Introduction
A prebiotic is defined as ‘a selectively fermented ingredient that allows specific changes, in the gastrointestinal (GI) microbiota that possess benefits upon host well-being and health’ Gibson and Roberfroid, 1995; Tzortzis et al., 2004). Interest in prebiotics has increased over the past few years. Beneficial prebiotics can modify gut function by targeting bacteria, which are already located in the large intestine. Oligosaccharide prebiotics have been recognized as beneficial dietary adjuncts and play critical role in regulating the colonic microbiota (Fuller and Gibson, 1998; Rabiu et al., 2001). Oligosaccharides are carbohydrates that composed with three to ten sugars connected by glycosidic bonds. Potential oligosaccharide prebiotics can be categorized according to their degree of polymerization and their chemical characteristics, and include isomalto-oligosaccharides, manno-oligosaccharides, pectic-oligosaccharides, xylo-oligosaccharides, fructo-oligosaccharides (FOS), and galacto-oligosaccharides (GOS) (Macfarlane et al., 2006; Macfarlane et al., 2008; Olano-Martin et al., 2003).
GOS are especially applicable to human nutrition as they are structurally similar to a variety of complicity of structures in human breast milk (Intanon et al., 2014; Sangwan et al., 2011). The presence of GOS in human milk supports the establishment of microbiota in the GI tract of newborn, breastfeeding infants (Gopal et al., 2001). GOS are usually synthesized from lactose by β-galactosidases produced by yeast, fungi, or bacteria, and have complex structures which include a variety of glycosidic bonds (Gobinath and Prapulla, 2014; Rabiu et al., 2001). These substrates function as prebiotics by supporting the growth of health-promoting microorganisms such as Bifidobacterium and Lactobacillus (Andersen et al., 2011; Davis et al., 2011; Garrido et al., 2013). Additionally, GOS are resistant to gastric acid and are poor substrates for hydrolytic enzymes in the upper digestive tract. Many in vitro studies have reported that Bifidobacterium and Lactobacillus strains can utilize GOS (Gopal et al., 2001; Smart et al., 1993; Yanahira et al., 1995). Despite interest in the use of GOS as a prebiotic, the mechanisms underlying its utilization by probiotics during fermentation are poorly understood, and our knowledge about the bioactive factors arising from synbiotic interactions between GOS and probiotics is limited. In addition, the potential of milk-derived GOS as a prebiotic substrate has not been sufficiently studied.
Probiotic lactic acid bacterial strains from the GI tract can survive in and colonize the small intestine and have a beneficial impact on host health (Forestier et al., 2000). Lactobacillus rhamnosus 4B15 was reported to have higher bioactive properties such as higher anti-oxidative activity, repression of α-glucosidase activity, cholesterol-reducing activity, and less production of nitric oxide (NO) compared to the other strains (Oh et al., 2018). In addition, 4B15 is known to inhibit the release of inflammatory cytokines including TNF-α, IL-6, IL-1β, and IL-10 and impacts immune health by modulating pro-inflammatory cytokines (Oh et al., 2018).
The aims of our study were optimization of the formation of GOS-enriched skim milk (GSM) during lactose hydrolysis by β-galactosidase, and evaluation of prebiotic effect of GOS after incubation. In addition, we made fermented GSM (FGSM) by selecting a Lactobacillus strain with probiotic potential and then determining its fermentation characteristics and functionality, evaluating cell counts, pH, antioxidant properties, and analyzing organic acids and bioactive peptides. The ultimate purpose of the present research is the development of a novel synbiotic fermented milk.
Materials and Methods
GSM was manufactured through hydrolysis of skim milk (SM) treated with β-galactosidase (Maxilact® LGI 5000, DSM, Netherlands) under the following conditions: 37°C, using 0.1% (w/w) of enzyme. SM was obtained from Seoul Dairy Cooperative (Ansan, Gyeonggi, Korea).
High-performance anion exchange chromatography with pulsed amperometric detection (HPAEC-PAD) in an ICS3000 Dionex system consisting of an SP-3000 gradient pump and ED-3000 electrochemical detector were employed for determining carbohydrate composition. Solvent temperature was 25°C on a CarboPac PA-1 analytical column (4×250 mm) connected to a CarboPac PA-1 (4×50 mm) guard column. Solvent A (15 mM NaOH) and solvent B (200 mM NaOH) were mixed to form the following gradient: 100% A from 0 to 15 min, followed by 0%-100% B from 15 to 45 min, kept constant for 2 min and then the column was washed for 10 min with 100% of solvent C (125 mM NaOH and 500 mM NaOAc) and re-equilibrated with the starting conditions.
For carbohydrate extraction, 0.2-gram aliquots of each sample were thoroughly mixed with 20 mL of distilled water and vortexed for 10 min. Carbohydrate extraction was performed twice using the same procedure. The mixture was separated by centrifugation at 5,000×g for 10 min, and the supernatant was filtered with a membrane filter (0.45-μm pore size). Finally, 25- L aliquots were employed using an autosampler system, and separations were carried out at a flow rate of 1 mL/min. Carbohydrates were quantified using standard curves produced from solutions with defined concentrations.
In this study, we selected a probiotic candidate as starter culture from among Bifidobacterium strains isolated from infant feces. After weighing, the feces homogenized in saline, and resuspended. Aliquots of the diluted sample were plated on Bifidobacterium selective (BS) agar (Kisan Bio, Seoul, Korea) and incubated at 37°C for 72 h under anaerobic chamber system. Strains were isolated and then purified using the plate-streak method on BS agar. BS agar is the medium for the selective isolation of bifdobacteria composed of beef extract, liver extract, yeast extract, proteose peptone, tryptone, soy peptone, soluble starch, glucose, dipotassioum phosphate, monopotassium phosphate, magnesium sulfate, sodium chloride, manganese sulfat, L-cysteine, ferrous sulfate, polysolbate 80, sodium propionate, paromomycin sulfate, meomycin, lithium chloride, and agar. The probiotic potentials of isolates were evaluated in terms of acid tolerance, bile tolerance, bacterial attachment, antibacterial activity, and cholesterol-lowering ability (data not shown). From the results, we selected four Bifidobacterium strains (B. infantis 5R08, B. longum 5R10, B. animalis 3B13, B. longum 1R09). A commercial strain, Bifidobacterium animalis subsp. lactis BB-12 (BB12), was employed for the determination of prebiotic effect.
For the determination of prebiotic effect, GSM was incubated with the five selected Bifidobacterium strains 5R08, 5R10, 3B13, 1R09, and BB12 (approximately 107 CFU/mL) at 37°C for 24 h. After 24 h of incubation, GOS contents in FGSM were analyzed using the above-mentioned methods. This study was approved by institutional review board of Samsung Medical Center (IRB No. 2017-08-040).
GSM and SM were inoculated with 3% suspensions of L. rhamnosus 4B15 (approximately 107 CFU/mL). The mixtures were incubated at 41°C for 24 h. All samples were lyophilized and stored at −80° until analysis.
In order to determine viable cell counts and pH in FGSM, 4B15 was enumerated on MRS at 37° for 72 h. Changes in FGSM pH were measured after calibration of the pH meter with standardized buffer solutions of pH 4.0, 7.0, and 10.0 (Fisher Scientific).
The antioxidant activity of FGSM was performed based on α-diphenyl-β-picrylhydrazyl (DPPH) radical scavenging activity, ferric reducing/antioxidant power activity (FRAP), and 2,2′-azino-bis (3-ethylbenzothiazoline-6-sulphonic acid) (ABTS) radical scavenging activity, using the described method by Oh et al. (2013).
The ability of FGSM to inhibit 3-hydroxy-3-methylglutaryl-CoA reductase (HMGR) was measured using an HMG-CoA reductase assay kit (Sigma-Aldrich, St. Louis, MO, USA). The HMG-CoA reductase inhibition assay was accessed by previous established procedure by Oh et al. (2014).
Milk peptide extraction by fermentation was conducted using the previous protocol by Oh et al. (2016). For analyzing peptide profiling, peptide extracts were resuspended with an equal volume of matrix solution (HCCA) and 1 μL was loaded onto an MTP SmallAnchor 384 BC target. MALDI-TOF/MS experiments were carried out using a Bruker Autoflex (Bruker Daltonics, Bremen, Germany) equipped with a nitrogen laser (337 nm).
All data in this study were indicated as means±SD. Statistical significance of the differences between the groups was confirmed by the methods of Duncan’s multiple range tests, and SAS software (version 9.2; SAS Institute Inc., Cary, NC) was employed to analyze all statistical tests. Differences with p<0.05 were considered significant.
Results
An optimization of the enzymatic synthesis was carried out to obtain the maximum GOS contents, with reaction time as the principal factor examined. To determine the influence of reaction time on GOS production, experiments were performed at 37< with an enzyme concentration of 0.1% (w/w).
Fig. 1 shows the HPAEC-PAD carbohydrate profile obtained when the maximum GOS concentration is reached. The presence of 8 products was identified. Peaks 1, 2, and 5 correspond with galactose (Gal), glucose (Glc), and lactose (Lac), respectively. In addition, three GOS were identified in the chromatogram including 6-galactobiose (peak 3, β-D-Gal-(1→6)-D-Gal), allolactose (peak 4, β-D-Gal-(1→6)-D-Glc), and 6-galactosyllactose (peak 7, D-Galp-(16)-D-Lac) as a result of transgalactosylation catalyzed by the enzyme. 4-galactobiose (peak 6, β-D-Gal-(1→4)-D-Gal) and 3-galactosylglucose (peak 8,β-D-Gal-(1→3)-D-Glc) were also identified. Collectively, our data suggest that the catalyzed transgalactosylation reaction produces GOS with various linkages such as β1→3, β1→4, and β1→6. The GOS produced mainly contained β1→6 linkages.
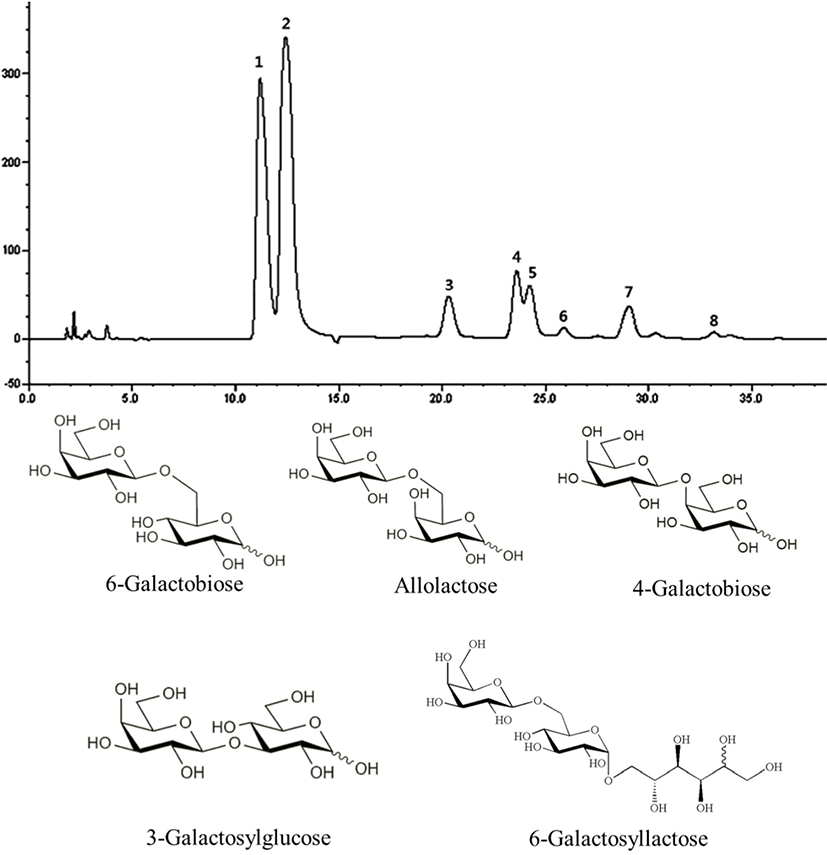
Fig. 2 and Table 1 show lactose and GOS contents during the time course of reaction, with lactose contents decreasing from 4.35±0.02 to 0.00 g/100 g over a period of 5 h. After 1 h, residual lactose contents had decreased by 98.16%, and maximum GOS yields (16.32%) had been reached. After 1 h of reaction, total GOS content was 0.71±0.01 g/100 g, which amounted to 14.67% of the total carbohydrate content. GOS in GSM was mainly composed of 6-galactosyllactose (0.23±0.00 g/100 g), followed by 6-galactobiose (0.18±0.00 g/100 g) and allolactose (0.17±0.00 g/100 g). Taking time, economic efficiency, GOS content, and lactose content into account, we treated SM with 0.1% (w/w) enzyme for 1 h to produce GSM.
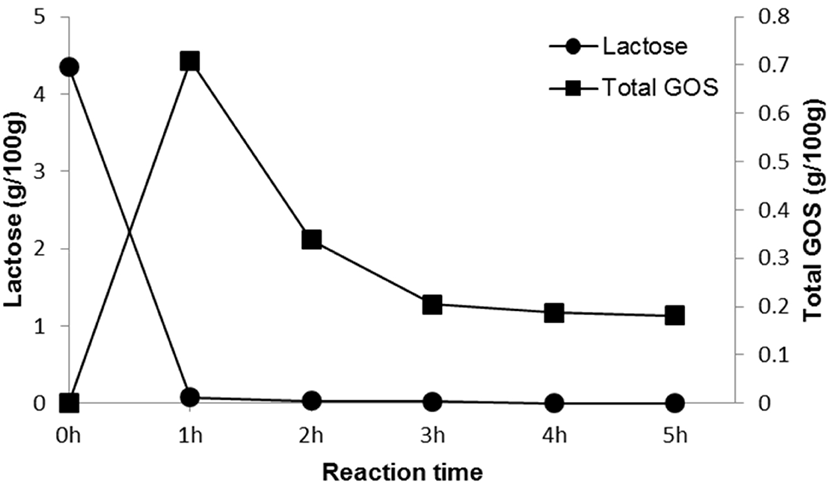
The GOS content of GSM after 24 h of incubation using the five selected Bifidobacterium strains is presented in Fig. 3. In this study, 6-galactobiose, allolactose, 4-galactobiose, 6-galactosyllactose, and 3-galactosylglucose were measured. After 24 h, the contents of 6-galactobiose in GSM incubated by strains 5R08, 5R10, 3B13, 1R09, and BB12 were 0.18±0.01, 0.17±0.01, 0.17±0.00, 0.18±0.00, and 0.17±0.00 g/100 g, respectively. The amount of 6-galactobiose was decreased by 17.92% (5R10) compared to the initial concentration in GSM, indicating that 6-galactobiose was utilized by Bifidobacterium strains during incubation. The amount of allolactose in GSM incubated by 5R08, 5R10, and 1R09 did not decrease significantly compared to its initial level in GSM. However, allolactose was decreased by 16.64% and 34.17% in GSM incubated by strains 3B13 and BB12, respectively. 4-galactobiose levels also decreased after fermentation, especially in GSM incubated by 5R08, with a 67.77% decrease compared to initial GSM levels. After 24 h, 6-galactosyllactose levels in GSM were similar to its initial level in GSM, with no significant difference except for in the case of 3B13 and BB12. Especially, 6-galactosyllactose contents in GSM incubated by BB12 increased compared to the initial 6-galactosyllactose contents in GSM. Additionally, after 24 h the 3-galactosylglucose contents of GSM incubated by strains 5R08, 5R10, 3B13, 1R09, and BB12 decreased by 22.67%, 3.38%, 52.65%, 13.23%, and 64.95%, respectively. The highest utilization rates of 3-galactosylglucose were found in GSM incubated with BB12. Collectively, the data showed that total GOS content in GSM decreased after 24 h of incubation by all five of the selected Bifidobacterium strains. Strains 3B13 and BB12 utilized the most GOS during incubation. From the results of this study, we confirmed that GOS can be utilized by probiotics, and determined the prebiotic effect of GOS.
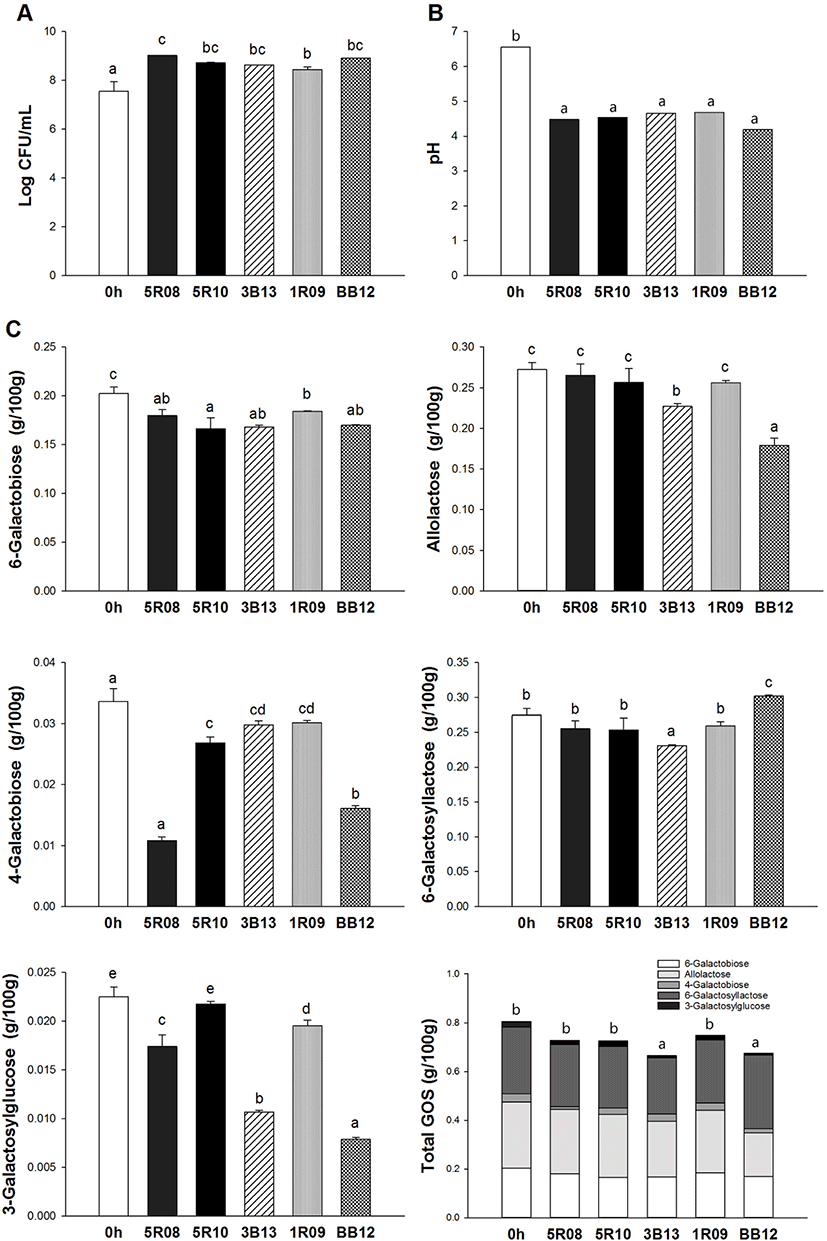
To determine the fermentation characteristics of GSM, we evaluated growth kinetics and pH during fermentation by the potentially probiotic strain 4B15 (Table 2). As a control, the viable cell counts in fermented SM (FSM) were measured at 8.24±0.02 Log CFU/mL after 24 h of fermentation. The pH of FSM had decreased slightly by the end of fermentation. On the other hand, the viable cell counts in FGSM (9.11±0.01 Log CFU/mL) were higher than that in the FSM. Additionally, the pH of FGSM decreased to 3.85 at the end of fermentation.
Fermentation time | SM | GSM | ||
---|---|---|---|---|
0 h | 24 h | 0 h | 24 h | |
Viable cell counts (Log CFU/mL) | ||||
4B151) | 7.52±0.08a | 8.24±0.02b | 7.60±0.03a | 9.11±0.01c |
pH | ||||
4B151) | 6.61 | 5.82 | 6.57 | 3.85 |
Carbohydrate contents in GSM fermented with 4B15 for 24 h are summarized in Table 3. As shown in Table 3A, after 24 h of fermentation, galactose and glucose contents decreased by 12% and 52% relative to their initial levels in unfermented GSM, respectively. Strain 4B15 utilized the most glucose during fermentation. On the other hand, lactose contents were not significantly different from their initial concentrations. The GOS content of FGSM during fermentation for 24 h using strain 4B15 is shown in Table 3B. The 6-galactobiose contents of FGSM at 0 and 24 h of fermentation with strain 4B15 were 0.22±0.00 and 0.20±0.00 g/100 g, respectively, with 6-galactobiose decreasing by 9.09% during fermentation. Of the allolactose originally in FGSM, 0.24±0.01 g/100 g was utilized, with only 8.33% being metabolized after 24 h of fermentation. With regard to 4-galactobiose and 3-galactosylglucose, there were no significant changes in concentration after 24 h of fermentation. Contents of 6-galactosyllactose decreased by 7.14% compared to unfermented GSM. Lastly, considering the total GOS content of FGSM, only 9.83% of GOS was utilized during fermentation and we confirmed that almost all GOS remained in FGSM.
Collectively, our data suggest that despite the completion of the fermentation, most of the GOS remained unused in the fermented products. This suggests that both probiotics and residual GOS are present following fermentation, which is beneficial to bioactive functionality.
Antioxidant activities were evaluated using DPPH, ABTS, and FRAP bio-assays. Specifically, the DPPH assay measured hydrogen-donating activity, the ABTS assay examined cation-scavenging activity, and the FRAP assay measured the reducing power of ferric ions. The DPPH radical scavenging activities of SM, GSM, FSM, and FGSM are described in Fig. 4A. FGSM had the highest radical scavenging activity (53.17%), followed by FSM (41.00%), SM (14.78%), and GSM (8.69%). To assess another mechanism of antioxidant activity, the ABTS assay was performed (Fig. 4B). FGSM had profound radical scavenging activity (32.36%), followed by FSM (27.49%), SM (13.46%), and GSM (12.03%). After 24 h of fermentation, the ABTS radical scavenging activities of SM and GSM increased by 104% and 169%, respectively, compared to their initial states. The ABTS and DPPH assays showed similar patterns of radical scavenging activities. FSM and FGSM activities were higher than those of SM and GSM, with increases equivalent to 290.08 and 772.58 ̜M FeSO4·7H2O, respectively (Fig. 4C). FGSM showed the highest reducing power, 3.59 times higher than those of GSM. SM, GSM, FSM, and FGSM in their initial states, as determined by HMGR inhibition activity (Fig. 4D). 3-Hydroxy-3-methylglutaryl-CoA reductase is the key factor in the mevalonate pathway, which converts cholesterol from HMG-CoA in the hepatic system (Oh et al., 2013). SM and GSM were significantly different at 0 h, with HMGR inhibition rate of GSM 10 times higher than that of SM. This indicated that the addition of GOS affects HMGR inhibition activities. In addition, FSM and FGSM increased HMGR inhibition rates (by 6.59% and 19.83%, respectively) compared with initial rates in SM and GSM, with FGSM showing the highest HMGR inhibition activity (3.65 times higher than GSM at 0 h).
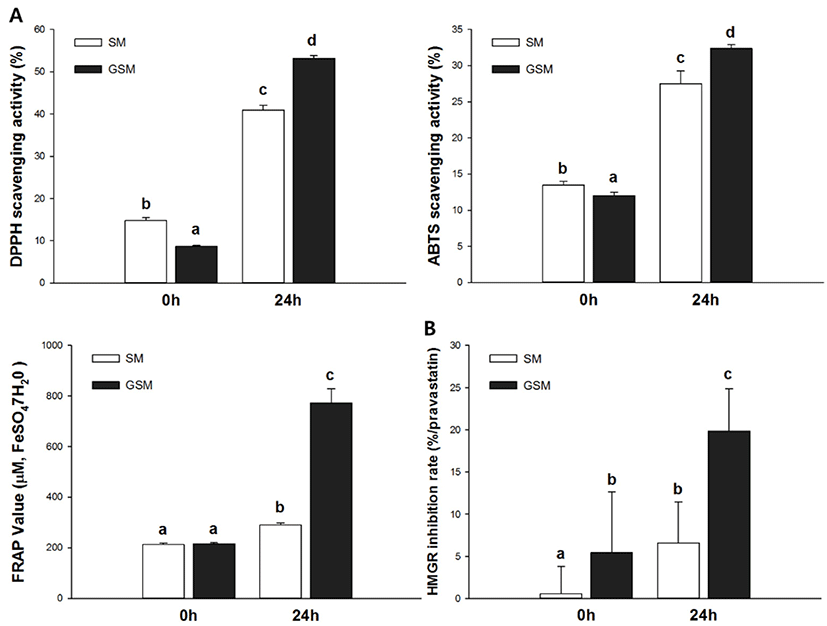
A featured peptide analysis of FGSM was performed by direct MALDI-TOF/MS/MS in the m/z range from 700 to 3,500 Da. As shown in Table 4 for peptide profiling from FGSM, thirty-nine peptide fragments were identified in FGSM. Most peptides derived from β-casein as well as αs1-casein, αs2-casein, and κ-casein. A total of thirty-one peptides originating from β-casein were identified, and C-terminal β-casein fragments (f199–209, f197–209, f195–209, f194–209, and f193–209) were identified in FGSM. In addition, β-casein-derived peptides f112–119, f173–182, f176–187, f78–93, f104–119, and f101–119 were only observed in FGSM. Five peptides originated from αs1-casein were identified. N-terminal αs1-casein fragments (f1–8, f1–9, and f1–17), along with a novel peptide fragment (f179–192) were identified. In the case of αs2-casein, only one peptide fragment (f101–114) was identified. Additionally, two peptides originating from κ-casein were identified, one of which, peptide κ-casein f54–60, was found only in FGSM.
m/z | Position | Sequence | References |
---|---|---|---|
αS1-Casein | |||
1012 | 1–8 | RPKHPIKH | Pisanu et al. (2015) |
1140 | 1–9 | RPKHPIKHQ | Albenzio et al. (2017) |
1219 | 25–35 | VAPFPEVFGKE | Padghan et al. (2017) |
1505 | 179–192 | FSDIPNPIGSENSE | |
1991 | 1–17 | RPKHPIKHQGLPQEVLN | Wu et al. (2013) |
αS2-Casein | |||
1650 | 101–114 | QGPIVLNPWDQVKR | Pisanu et al. (2015) |
β-Casein | |||
826 | 199–206 | GPVRGPFP | Zhao et al. (2016) |
976 | 112–119 | PKYPVEPF | |
1001 | 60–68 | YPFPGPIPN | Bottari et al. (2017) |
1057 | 85–93 | PPFLQPEVM | Oh et al. (2017) |
1100 | 59–68 | VYPFPGPIPN | Eisele et al. (2013) |
1126 | 173–182 | VPQKAVPYPQ | |
1151 | 145–154 | HQPHQPLPPT | Fialho et al. (2018) |
1151 | 199–209 | GPVRGPFPIIV | Rodriguez-Figueroa et al. (2012) |
1157 | 132–141 | NLHLPLPLLQ | Sommella et al. (2016) |
1265 | 198–209 | LGPVRGPFPIIV | Ha et al. (2015) |
1282 | 144–154 | MHQPHQPLPPT | Chenoll et al. (2016) |
1351 | 109–119 | MPFPKYPVEPF | Padghan et al. (2017) |
1364 | 197–209 | VLGPVRGPFPIIV | Ha et al. (2015) |
1382 | 145–156 | HQPHQPLPPTVM | Ul Haq et al. (2015) |
1393 | 194–206 | QEPVLGPVRGPFP | Villegas et al. (2014) |
1414 | 176–187 | KAVPYPQRDMPI | |
1431 | 132–143 | NLHLPLPLLQSW | Padghan et al. (2017) |
1452 | 81–93 | PVVVPPFLQPEVM | Xue et al. (2018) |
1469 | 143–154 | WMHQPHQPLPPT | Oh et al. (2016) |
1481 | 108–119 | EMPFPKYPVEPF | Thomas et al. (2016) |
1513 | 144–156 | MHQPHQPLPPTVM | Cossais et al. (2017) |
1556 | 193–206 | YQEPVLGPVRGPFP | Ha et al. (2015) |
1718 | 194–209 | QEPVLGPVRGPFPIIV | Ha et al. (2015) |
1782 | 78–93 | TQTPVVVPPFLQPEVM | |
1851 | 145–160 | HQPHQPLPPTVMFPPQ | Tellez et al. (2010) |
1874 | 105–119 | KHKEMPFPKYPVEPF | Kalyankar et al. (2013) |
1881 | 193–209 | YQEPVLGPVRGPFPIIV | Eisele et al. (2013) |
1971 | 104–119 | PKHKEMPFPKYPVEPF | |
1982 | 144–160 | MHQPHQPLPPTVMFPPQ | Tapal et al. (2016) |
2042 | 103–119 | APKHKEMPFPKYPVEPF | Mansor et al. (2013) |
2244 | 101–119 | AMAPKHKEMPFPKYPVEPF | |
κ-Casein | |||
1329 | 75–85 | ARHPHPHLSFM | Qian et al. (2011) |
1458 | 54–65 | QFLPYPYYAKPA |
Discussion
Several studies have reported that Bifidobacterium spp. is capable to metabolize a number of carbohydrates (O’Connell Motherway et al., 2013; Schell et al., 2002; Ventura et al., 2007) and over 50 different kinds of carbohydrases originated by bifidobacteria have been reported (O’Connell Motherway et al., 2013; Pokusaeva et al., 2011; van den Broek et al., 2008). In order to metabolize GOS, bacteria transport and then metabolize galactose, using specific glycosyl hydrolases, in central metabolic pathways (Garrido et al., 2013). In vitro, several intestinal bacteria, including bifidobacteria, have been shown to utilize GOS, and fecal bifidobacterial numbers have occasionally been found to increase after GOS consumption (Alander et al., 2001; Ito et al., 1993).
In the present study, we evaluated the prebiotic effect of GOS by analyzing its usage by five selected Bifidobacterium strains (5R08, 5R10, 3B13, 1R09, and BB12), finding that the five selected Bifidobacterium strains utilized all tested GOS (6-galactobiose, allolactose, 4-galactobiose, 6-galactosyllactose, and 3-galactosylglucose) during incubation. However, total 6-galactosyllactose increased after the end of incubations performed by strain BB12. This observation suggests that BB12 possesses different uptake systems for the utilization of GOS. These results indicated that Bifidobacterium strains were capable of metabolizing GOS during the incubation and demonstrated the potential of GOS as a prebiotic substitute.
Based on the verification of GOS’s prebiotic effects, we produced FGSM with the potentially-probiotic strain 4B15. Subsequently, we measured the fermentation characteristics of GSM, including growth kinetics and pH, during fermentation. FGSM had a lower pH than FSM at the end of fermentation, with 4B15 growing better in FGSM than in FSM by an order of magnitude.
We also analyzed the GOS content of FGSM after 24 h of fermentation. Although the total GOS contents of FGSM decreased, the decrease was comparatively minor. Only 9.83% of the total available GOS was metabolized during the FGSM fermentation. This indicated that FGSM has not only active Lactobacillus, which has potential bioactivity, but also substantial amounts of beneficial prebiotic GOS. GOS are excellent food additives that improve health benefits and support the growth of health-promoting microbiota in the gut environments (Garrido et al., 2013). They have reported to have a variety of properties benefiting health, contributing to intestinal barrier function improvement and stool improvement as well as, carcinogenesis, allergy alleviation and weight management (Lamsal, 2012).
In our experiments, we evaluated the enhanced antioxidant activity of the fermented product with or without GOS during fermentation. After 24 h of fermentation, GSM showed higher antioxidant activity than SM in three kinds of bioassay results including DPPH radical scavenging activity, ABTS radical scavenging activity, and FRAP values. Downregulation of cholesterol is related to the reduction of HMGR, as active compounds restrict synthesis of cholesterol in the liver by controlling critical enzymes (Crowell, 1999; Ghasemi and Taherpour, 2015). One important mechanism involves oligosaccharides diminishing blood cholesterol levels by binding bile acids in the intestines, thereby regulating lipid absorption, and increasing cholesterol removing and synthesis of new bile acid in the liver (Ghasemi and Taherpour, 2015; Ooi and Liong, 2010). Additionally, cholesterol metabolism is associated with synthesis of bile salt from cholesterol in the liver, and with restriction of the enzyme CYP7A1 (Fava et al., 2006; Saha and Reimer, 2014). In the present study, the HMGR inhibition rate of GSM was about 3 times higher than the HMGR inhibition rate of SM after 24 h of fermentation. These results corresponded with the results of the DPPH, ABTS, and FRAP assays in this study, and demonstrated a connection between antioxidant activity and HMGR inhibition.
Milk contains important proteins including casein and whey protein such as α-lactalbumin, β-lactoglobulin, and immunoglobulins. Milk proteins perform their biological activities not only directly, but also when processed through metabolism into various peptide forms. Although their biological activities vary, the majority of these proteins affect the functionality of the immune system in some way (Ebringer et al., 2008). Casein is processed into various bioactive peptides through reaction with proteolytic enzymes (Ebringer et al., 2008; Korhonen and Pihlanto, 2006). These bioactive peptides have different effects on the cardiovascular, digestive, and nervous systems (Ebringer et al., 2008; Korhonen and Pihlanto, 2006). Among them, featured oligopeptides derived from α- and β-caseins such as casomorphines, lactorphines, and casokinines are reconstructed from milk by metabolizing proteins from lactic acid bacteria (Ebringer et al., 2008; Oh et al., 2016). Peptides created by this reaction have two or more biological activities and include small peptides such as Val-Pro-Pro and Ile-Pro-Pro which have hypotensive effects (Ebringer et al., 2008; Huth et al., 2006; Seppo et al., 2003). In addition, some peptides originating from β-lactoglobulin, such as the peptide Ile-Ile-Ala-Glu-Lys, exert hypocholesterolemic effects (Ebringer et al., 2008; Nagaoka et al., 2001). In the current study, the FGSM produced with strain 4B15 showed improved antioxidant activity and HMGR inhibition. The enhancement of antioxidant activities and HMGR inhibition in FGSM can be attributed to the generation of peptides by 4B15 during fermentation step. Peptides originated from the fermentation of milk have been reported to have immunostimulatory, antitumor, antimicrobial, antihypertensive, and antioxidative activities, as well as ACE inhibitory activity (Ebringer et al., 2008; Miguel et al., 2006; Oh et al., 2016). Among the identified thirty-nine peptide fragments, six β-casein peptide fragments (f112–119, f173–182, f176–187, f78–93, f104–119, and f101–119), one αs1-casein peptide fragment (f179–192), and one κ-casein peptide fragment (f54–60) were only observed in FGSM.
The peptides specifically released from GSM after fermentation by 4B15 have been reported to have various bioactivities such as antimicrobial, ACE inhibitory, antioxidant, anti-cancer effects (Davis et al., 2011; Eisele et al., 2013; Pisanu et al., 2015; Tapal et al., 2016; Villegas et al., 2014). The newly isolated peptides in this study, FSDIPNPIGSENSE, PKYPVEPF, VPQKAVPYPQ, KAVPYPQRDMPI, TQTPVVVPPFLQPEVM and PKHKEMPFPKYPVEPF are expected to have health promoting effects, since the selected strains, 4B15 have been shown to enhance the anti-oxidation, anti-inflammation and cholesterol lowering, respectively (Oh et al., 2017; Oh et al., 2018). However, further studies are needed to fully understand their bioactivities.
In conclusion, we investigated the prebiotic potential of GSM. First, we evaluated the prebiotic effect of GSM through analysis of its utilization by Bifidobacterium strains (5R08, 5R10, 1R09, 3B13, and BB12) during incubation. From the results, we confirmed that GOS can be utilized by Bifidobacterium strains, and we determined the prebiotic effect of GSM. Second, we evaluated the fermentation characteristics and antioxidant capacities of GSM after fermentation with 4B15, a strain with probiotic potential. We confirmed that GSM stimulated the growth of 4B15, but was not completely consumed during fermentation, and that some remained in FGSM. We also confirmed that the antioxidant activity and HMGR inhibition rate of FGSM produced with 4B15 increased relative to that of the control after 24 h of fermentation, and that this was caused by metabolizing functional substrates such as organic acids and peptides. Finally, we found eight novel peptide fragments possessing potential bioactivities. In conclusion, we found that GSM can be used for potentiating prebiotics, and it enhances functional bioactivities through interaction with probiotics. Therefore, our results will contribute to the development of new dairy products with beneficial functionality. GSM may be applied not only in various dairy foods but also in the food industry. Adding to this, our findings contribute on the development of a new insight for synbiotic fermented milk.