Introduction
Meat has been recognized as a food source that provides essential human nutrients such as proteins (Hwang and Hong, 2020). However, the meat quality is degraded during distribution and storage, often due to microbial activity (You et al., 2020). Thus, lowering the storage temperature is necessary to extend the acceptable shelf life by reducing the rates of reactions that lead to deterioration, including microbial spoilage, lipid oxidation, and proteolysis (Pomponio and Ruiz-Carrascal, 2017; Stonehouse and Evans, 2015). Refrigeration is an effective method to delay food degradation, but this storage method can only extend the shelf life for a few days (Kang et al., 2020). Freezing is considered as an efficient storage method to preserve the freshness of meat. However, quality degradation of meat occurs due to ice crystals that are generated during the freezing process, resulting in the collapse of cells in the food leading to drip loss (Yun et al., 2021).
Supercooling treatment is a novel technique used to replace the freezing storage method, which is defined as lowering the temperature of a product below its freezing point without phase transition (Stonehouse and Evans, 2015). Although storage at deep freezing temperatures can extend the shelf life of pork loins, quality loss is inevitable due to the phase transition of the freezing process (Hong et al., 2009). Furthermore, the costs of frozen storage and thawing are additional concerns (Kang et al., 2020). Thus, supercooling storage has potential value for extending shelf life without structural damage and denaturation induced by ice crystal formation during the freezing process as well as satisfy the demands of customers, who regard foods stored at the refrigerated condition are fresher than frozen foods (Park et al., 2021a; Stonehouse and Evans, 2015). However, a supercooled product is theoretically unstable, and nucleation can occur spontaneously (Bédécarrats et al., 2010; Fukuma et al., 2012). Fukuma et al. (2012) detailed that vibration and temperature fluctuations can induce nucleation in supercooled foods.
Owing to the limitations in the achievement and maintenance of supercooling, a limited number of studies have been conducted on the supercooling storage of meats. Mok et al. (2017) combined pulsed electric fields and oscillating magnetic fields for supercooling storage of a chicken breast and extended the supercooled state for 12 h at −6.5°C, despite that conventionally cooled samples are frozen at this temperature. Without specific instruments for supercooling foods, Fukuma et al. (2012) reached supercooled state of fish using only temperature control. The authors employed stepwise cooling to achieve supercooling storage for 10 mm-thick fish slices (from yellow tail, flatfish, and red sea bream) in incubators. The set temperature was reduced by 0.5°C or 1.0°C per day and the fish slices were unfrozen in the range of −8.0°C to −3.5°C depending on the fish types and conditions, delaying the loss of texture. Furthermore, Kim et al. (2021) and Park et al. (2021b) reported that mackerel and hair tail preserved in supercooled storage using a stepwise algorithm maintained a 100% supercooled state. That research exploited stepwise algorithms that reduced temperature from −1.0°C to −2.0°C by 0.5°C every 18 h and repeated this cycle, considering the freezing point of fish. The researchers speculated that the kinetic energy was insufficient for ice crystallization because of the very slow rate of cooling, and that small molecules in the muscles may be able to depress the freezing point. Nevertheless, research on the supercooling of foods were mainly focused on the achievement and maintenance of supercooled state of foods and few studies have been conducted to for supercooling storage and the effects on the physicochemical properties of supercooled meat.
Therefore, this study was investigated to find the feasibility of supercooling storage of pork loins. A stepwise algorithm was applied for the temperature control method to accomplish supercooling storage of pork loins without any specific instruments such as electromagnetic fields. This algorithm was designed for reducing the cooling rate, achieving a lower storage temperature, inhibiting ice nucleation by repeating cycle, and consequently extending the shelf life of pork loins. After cooling treatments, pork loins were analyzed to physicochemical properties and microbial analysis for the investigation on quality of supercooled pork loin, compared to those of conventional refrigerated or frozen pork loins.
Materials and Methods
Pork loins were purchased at 48 h post-mortem from a local butcher shop, and cut into 30×30×30 (width×length×height) mm cubes. Total 48 samples were covered with kitchen wrap twice. To record the change in temperature in the samples, T-type thermocouples were applied to the surface of the samples, and the surface temperature of the samples was recorded every 1 min during cooling. A data logger (Data Acquisition-MX 100, Yokogawa, Tokyo, Japan) was used to confirm that the temperature of the samples was appropriate and ascertain whether the sample was frozen or not. Fresh pork loin was used as the control.
Each twelve samples were subjected to four types of cooling treatments. Each 6 samples were placed into an expanded polystyrene box (20 mm thickness) for physicochemical analysis after 8 and 16 days. Refrigeration treatment (3°C) was conducted in a refrigerator (R-F875HBSW, LG Electronics, Seoul, Korea) that was set at 3°C. Supercooling treatment was conducted in a refrigerator (K417SS13, LG Electronics, Seoul, Korea) controlled with a 4-day cycle stepwise algorithm. Initially, the temperature was set to −1°C and reduced by 0.5°C every 0.5 day four times. After reaching −3°C, the temperature was maintained at −3°C for 2 days. This algorithm was repeated every 4 days. Freezing treatments were performed at −3°C and −18°C. Freezing treatment was conducted using refrigerators (K417SS13, LG Electronics) set to −3°C and in a refrigerator (R-F875HBSW, LG Electronics) set to −18°C to compare the effect of the temperature between the supercooling and freezing treatments. The boxes were unpacked at the end of storage for physicochemical analysis. Frozen samples were thawed at 3°C for 0.75 days prior to analysis.
Fresh pork loin and treated pork loin cubes with the exudate removed using dry tissues were weighed. Drip loss was determined by calculating the difference in the weight of the fresh and treated samples and expressed with the following formula:
The samples were vacuum-sealed in plastic bags and heated in a water bath (BF-30SB, BioFree, Seoul, Korea) at 70°C for 30 min. The heated samples were cooled to ambient temperature for 30 min. Samples were weighed before and after cooking. The cooking loss for the pork loin samples was expressed using the following formula:
One gram of each sample was wrapped with absorbent cotton and placed in a centrifuge tube. After centrifugation using a centrifuge separator (1736R, Labogene, Seoul, Korea) at 3,000×g for 10 min at 4°C, the samples were weighed. The WHC of the pork loin samples was expressed as the ratio of the sample weight after centrifugation to the initial sample weight using the following formula:
Samples for which the cooking losses were measured were cut into 10×20×10 (width×length×height) mm cuboid shape. The shear force was measured using a texture analyzer (CT3 texture analyzer, AMETEK Brookfield, Middleborough, MA, USA), and the parameters were set as follows: Compression type, TA22 probe, TA/SBA fixture, test speed 2.5 mm/s, trigger load 5 g, and target value 10 mm.
Color parameters for each sample were determined using a colorimeter (CR-400 Chroma Meter, Konica Minolta, Osaka, Japan). The lightness (CIE L*), redness (CIE a*), and yellowness (CIE b*) values of the samples were measured after calibration with a white standard plate (CIE L*=96.79, CIE a*=+0.30, CIE b*=+1.67). The total color difference (ΔE) was calculated using the following equation for the difference between the fresh and thawed samples.
The pH of the samples was measured using a pH meter (Orion 3 Star, Thermo Scientific, Waltham, MA, USA). Five grams of the sample was homogenized with 45 mL of distilled water using a homo-mixer (CNHR 26, Bosch, Ljubljana, Slovenia) for 40 s.
The VBN content of the pork loin samples was determined according to Conway’s micro-diffusion method (Kim et al., 2020). Five grams of the sample was homogenized with 45 mL of distilled water using a slap-type homogenizer (WS-400, Shanghai Zhisun Equipment, Shanghai, China) for 180 s. The homogenate was filtered using Whatman No.1 filter paper (GE Healthcare Life Science, Sheffield, UK). After filtering, 1 mL of the filtrate was placed in the outer section of a Conway dish with a mixture of 1 mL of 0.01 N H3BO3 and 100 μL of Conway solution (mixture of 0.066% methyl red in ethanol and 0.066% bromocresol green in ethanol) was dropped into the inner section of the dish. Then, 1 mL of 50% K2CO3 was added to the outer section of the dish. The Conway dish was closed and incubated at 37°C for 2 h, then titrated using 0.02 N H2SO4 until the Conway reagent changed to a red color. The VBN content was determined following the addition of 0.02 N H2SO4 to the inner section of the Conway dish. The VBN values were calculated using the following formula:
where a is the titration volume for the sample (mL), b is the titration volume for the blank (mL), f is the factor for H2SO4, S is the weight of the sample (g), and c is the dilution amount.
The TBARS in each sample were measured according to the procedure described by Park et al. (2021a). Five grams of the sample was homogenized with 45 mL of distilled water for 60 s. The homogenate was filtered using Whatman No.1 filter paper (GE Healthcare Life Science). The filtrate (0.5 mL) was transferred to a test tube and 4.5 mL of TBA solution (0.25 N HCl, 15% trichloroacetic acid, and 0.375% TBA reagent) was added. The tube was heated (95°C for 15 min) in a water bath (BF-30SB, BioFree), cooled to room temperature, and centrifuged at 3,000×g at 4°C for 10 min. Then, 200 μL of the supernatant was placed in a 96-Well plate (SPL Life Science, Pocheon, Korea) and the absorbance was measured using a spectrophotometer (MultiskanTM GO UV/VIS, Thermo Fisher, Waltham, MA, USA) at 535 nm. The TBA values of the samples are presented as mg malondialdehyde/kg (mg MDA/kg).
One gram of sample was collected from the surface of the pork loin sample and homogenized with 9 mL of sterilized 0.85% NaCl solution for 1 min using a slap-type homogenizer (WS-400, Shanghai Zhisun Equipment). The supernatant was diluted by serial dilution and 1 mL of diluted solution was placed on ready-to-use Petrifilm aerobic count plates (3M Petrifilm, 3M, Saint Paul, MN, USA) and incubated for 2 days at 37°C in an incubator (SI-600R, Jeio Tech, Seoul, Korea). The colonies were counted and were expressed as Log colony-forming units per 1 g of sample (Log CFU/g) to obtain the TAC.
Results and Discussion
For stable supercooling storage, a stepwise cooling algorithm was designed considering three main parameters: 1) minimization of temperature deviation during storage, 2) suitable setting of the lowest temperature at the end of one cycle for the type of food, and 3) returning to the initial temperature of one cycle at the end point (Fig. 1). From our previous experiment (Lee, 2020), the fluctuation of temperature was more than 0.3°C, which is difficult to supercool during the storage period. The second parameter was set to the lowest suitable temperature. According to Kim (2021), the lowest temperature of chicken breast adopted for the stepwise cooling algorithm was set at −2.5°C for 0.75 day (18 h), which can preserve the supercooled state without phase transition. Although the samples maintained a supercooled state at the lowest temperature owing to the small temperature fluctuations, the supercooled state was thermodynamically unstable. Therefore, to prevent unexpected cancellation of supercooled conditions, it is necessary to limit the exposure time of food to the lowest temperature and return to the initial temperature at the start of the second cycle.
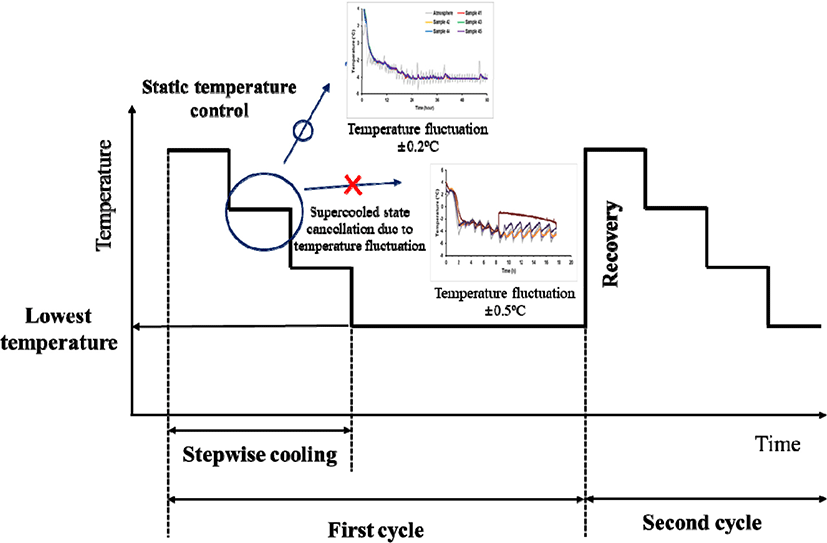
The temperature curves for the refrigeration, supercooling, and freezing treatments of pork loin samples are shown in Fig. 2. Refrigeration treatment was conducted at 3°C, and all pork loin samples reached 3°C after 0.08 day (2 h) of storage (Fig. 2A). Supercooling treatment was conducted by employing the stepwise algorithm (Fig. 2B), and the mean target temperature during storage was about −2.4°C, which was below the initial freezing temperature of −0.6°C for the pork loin samples (Kim and Hong, 2016). Thus, in this study, the stepwise algorithm was regarded as supercooling for pork loin. The temperatures of the pork loin samples were slowly cooled in stages and maintained at −3°C for 2 days (48 h). Then, the target temperature was raised to −1°C and the stepwise algorithm was repeated. While repeating the program, the sample temperatures followed the target temperature below 0.5°C. In 6-8th day, the sample temperatures were maintained in the range of −3.5°C to −3.2°C without phase transition. Ten of twelve samples retained the supercooled state for up to 16 days of storage, and no ice was detected on the surface of the supercooled pork loin samples at the end of storage. The center temperatures of the pork loin samples frozen at −3°C reached the target temperature after 0.10 day (2.5 h) and generally remained within the range of −3.5°C to −3.0°C (Fig. 2C). The sample temperature reached −18°C after 0.29 day (7 h) in a refrigerator set to −18°C (Fig. 2D). These samples were stored at −18°C to −19°C for 16 days.
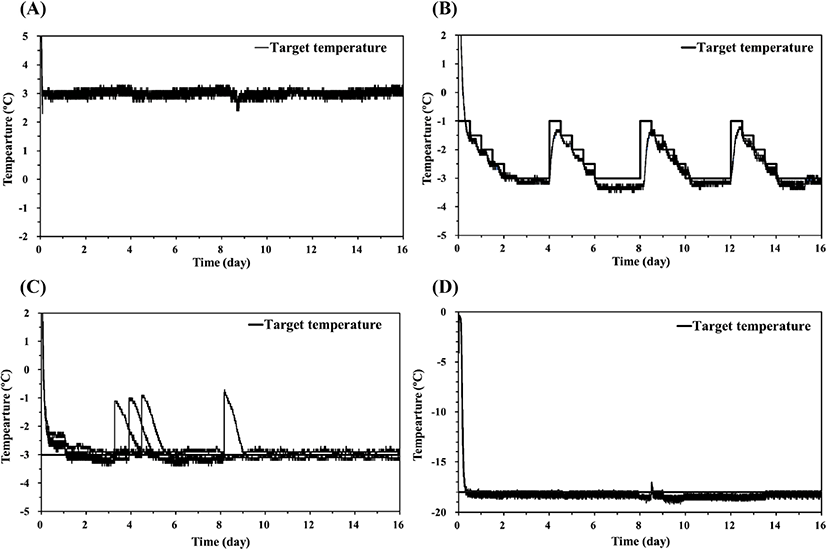
The drip loss for pork loin samples subjected to the various treatments and storage periods are presented in Table 1. Drip not only affects meat quality, such as weight and nutrition, but also plays a role as a good substrate for microbial growth (den Hertog-Meischke et al., 1997). Refrigerated pork loin samples showed approximately 10.5% drip loss, regardless of the storage period. The drip loss of supercooled pork loin sample increased during storage. After 8 days of storage, the supercooled pork loin sample had the lowest drip loss (p<0.05) at 8.24%, which increased to 9.44% after 16 days of storage. The two freezing treatments exhibited different patterns. There was no significant difference between pork loin samples frozen at −18°C and −3°C (p>0.05) after 8 days of storage. The drip loss on day 16 for samples frozen at −3°C and −18°C was 10.49% and 6.74%, respectively.
Extrinsic factors such as freezing and thawing processes induce a larger amount of drip from meat due to ice crystallization, leading to rupture of the fiber membrane (Benjakul and Visessanguan, 2010; Lambert et al., 2001; Turan et al., 2003). Many studies have analyzed factors affecting thawing loss, such as post-mortem aging, frozen storage, and thawing conditions (Añón and Calvelo, 1980; Kondratowicz et al., 2006; Kondratowicz et al., 2008). Thus, quickly lowering the temperature of meat can avoid various metabolic processes related to drip loss (Huff-Lonergan and Sosnicki, 2002), and low-temperature storage of meat is necessary to reduce the overall quality loss after slaughter. Duun and Rustad (2008) observed that pork roasts stored at −2°C had less drip loss than samples stored at 3.5°C and −36°C. Similarly, supercooled chicken breast had lower drip loss compared to refrigerated and partially frozen samples after 12 h of storage (Mok et al., 2017).
In this study, supercooling treatment was regarded as superior for the reduction of drip loss compared to conventional refrigeration and freezing, as the drip loss was no higher after 16-day storage. Consequently, it was concluded that supercooling and freezing at lower temperatures are advantageous for drip loss in meat.
The cooking losses for pork loin samples subjected to various treatments and storage periods are presented in Table 1. Cooking loss is the weight loss of the released liquid and the hydrophilic content during cooking (Aaslyng et al., 2003). The cooking loss for fresh pork loin samples was approximately 30.9% and significantly increased with storage (p<0.05), ranging from 35.5% to 38.0%. There was no marked difference in cooking losses for pork loin samples after eight days of storage, regardless of the cooling treatment (p>0.05). On the 16th day, the cooking losses for pork loin samples did not increase, regardless of treatment, compared to the losses after eight days (p>0.05). However, the cooking loss of supercooled pork loin sample was 35.5% which was the lowest value among the treatment groups and although the difference was small.
Cooking meat leads to protein denaturation which deprives the protein structure of entrapped water due to the loss of capillary forces (Aaslyng et al., 2003). Vieira et al. (2009) mentioned that chemically bound water in meat is released by melting fat and denaturing proteins during cooking. Several studies report that the moisture loss caused by cooking is not vastly different between unfrozen meat and frozen and thawed meat (Leygonie et al., 2012; Vieira et al., 2009). In contrast, Kim et al. (2015) reported that the cooking loss of frozen/thawed pork was higher than that of fresh pork, regardless of various freezing and thawing processes, due to ice crystallization and recrystallization. There have been irreconcilable reports regarding cooking loss in meat stored under refrigeration. Straadt et al. (2007) observed an increase in the tendency of cooking loss for pork stored at 4°C for four days, with a constant loss after this period for up to 14 days due to the disintegration of damaged fibers during aging. Furthermore, Lu et al. (2019) found higher cooking loss in M. longissimus lumborum muscles from Chinese yellow cattle stored via superchilling at −4°C than in those stored under conventional refrigeration (2°C) and freezing (−18°C). They attributed this result to severely damaged cell membranes during superchilling storage. It was noted that supercooling treatment for unfrozen pork loin samples was advantageous for cooking loss within 16 days of storage.
The WHC values of pork loin samples subjected to various treatments and storage periods are presented in Table 1. WHC is the ability of the muscle to retain water from loss to external forces (Huff-Lonergan and Sosnicki, 2002). The loss of WHC is associated with the destruction of muscle fiber structures and protein denaturation and/or modification, which shrinks myofibrils (Huff-Lonergan and Sosnicki, 2002; Savage et al., 1990). The WHC of fresh pork loin sample was 85.1%. On day 8 of storage, the WHC values for all pork loin samples decreased. However, there were no significant differences compared to that of the 0 day and other treated samples (p>0.05), with WHC above 80%. However, unfrozen (refrigerated and supercooled) and frozen pork loin samples had different WHC after 16 days of storage. The WHC of unfrozen pork loin samples was above 83% and was not markedly different from that of fresh pork loin samples (p>0.05). This result is consistent with that obtained by Kristensen and Purslow (2001). The authors reported that WHC of longissimus muscle was maintained after 10 days during aging process (stored at 4°C), though the WHC initially increased and decreased. Other research also reported about these phenomena, which showed the WHC of non-frozen meat did not decline (Joo et al., 2008; Miller et al., 2007). Kristensen and Purslow (2001) attributed to the proteolysis of cytoskeletal proteins and weight loss of the muscle joints caused by water loss. In contrast, frozen pork loin samples had significantly lower WHC (p<0.05), below 80%, although there was no obvious difference based on the freezing temperature (p>0.05). This may be ascribed to the structural changes in muscle proteins induced by ice crystals due to freezing and thawing (Huff-Lonergan and Lonergan, 2005; Straadt et al., 2007). Thus, refrigeration and supercooling treatments were regarded as beneficial for sustaining muscle fiber structures within 16 days based on the different WHC trends between frozen and unfrozen pork loin samples.
The shear forces of pork loin samples subjected to various treatments and storage periods are presented in Table 1. Meat tenderness is an important property for consumers (Pomponio et al., 2018). The shear force of fresh pork loin sample was 6,412 g, which was reduced by the different treatments and storage periods. In general, unfrozen pork loin samples had lower shear force. After eight days of storage, unfrozen samples had shear forces under 4,500 g, whereas those of frozen samples were approximately 4,800 g. There were no significant differences among the treatment groups (p>0.05). On the 16th day, the shear force of refrigerated pork loin samples was approximately 4,300 g and that of supercooled samples was approximately 3,900 g, which was the lowest value obtained.
A decrease in the shear force of pork under refrigeration is consistently reported due to initial tenderization within the first week after slaughter (Juárez et al., 2009; Juárez et al., 2011; Rees et al., 2002) followed by a steady state (Xiong et al., 2006). This decrease in shear force may have been caused by the degradation of muscle fibers and major myofibrillar proteins by enzymatic proteolysis related to calpains and cathepsins (Huff Lonergan et al., 2010). The calpain system is a group of several proteins. μ- and m-calpains are associated with the proteolysis of myofibrillar proteins, which are calcium dependent and activated during autolysis (Goll et al., 2003; Huff Lonergan et al., 2010; Pomponio et al., 2008). Cathepsins are peptidases that exist in lysosomes and tenderize meat after leakage through the disintegration of lysosome membranes caused by accumulated lactic acid (Ertbjerg et al., 1999). Thus, temperature is a major factor in physiological proteolysis and tenderization (Huff Lonergan et al., 2010; Pomponio and Ertbjerg, 2012). Pomponio et al. (2018) also observed a decreasing tendency in the Warner-Blatzer shear force of pork loins under refrigeration and superchilling storage (−1°C). Conversely, freezing treatment led to an increase in the shear force, which was above 5,000 g. The pork loin frozen at −3°C had a shear force of approximately 5,500 g, which was the highest value compared to that of the 0 day. Consequently, it was concluded that supercooling treatment resulted in the tenderization of pork loin samples.
The colors of pork loin samples subjected to various treatments and storage periods are presented in Table 2. The CIE L* value of fresh pork loin sample was 43.96 and the storage temperature significantly affected the CIE L* values. The CIE L* of refrigerated pork loin sample increased to above 55 after 16 days of storage. However, the supercooling treatment maintained the CIE L* value of pork loin sample on the 16th day. The CIE a* value of fresh pork loin samples was 6.64 and the refrigerated pork loin samples showed a decrease in the CIE a* value to 5.83 after eight days of storage, and eventually to 5.55 (p<0.05). In contrast, supercooling and freezing treatments did not lead to a change in the CIE a* of pork loin samples during the storage period (p>0.05). The CIE b* values increased significantly by the different treatments and storage periods (p<0.05). The CIE b* of fresh pork loin samples was 2.98 and increased to 4.95–5.78 after eight days of storage. However, the CIE b* value did not change substantially within the 16 days of storage in all treatment groups. Similarly, the total color difference (ΔE) of pork loin samples also varied. After eight days of storage, there were no significant differences among the treatment groups (p>0.05). However, after 16 days of storage, the total color difference for refrigerated pork loin samples increased to 11.75, which was the highest value (p<0.05).
Color is considered as an index of meat quality, and bright red is regarded as an indicator of freshness by consumers (Mancini and Hunt, 2005; Ye et al., 2020). Martin et al. (2013) indicated that a higher discoloration rate was induced by a higher storage temperature for meat and the color change was alleviated at sub-zero temperatures (Gill and McGinnis, 1995). Hence, lower storage temperatures enhance the color stability of meat during storage (Buys et al., 1993). This was attributed to the higher oxymyoglobin content and lower metmyoglobin content in supercooled steak than in those stored at 5°C, indicating that supercooled meat was redder than those stored under refrigeration conditions (Jeremiah and Gibson, 2001). These findings demonstrate that refrigeration results in discoloration of pork loin samples after 16 days of storage, resulting in the highest CIE L* and the lowest CIE a* and CIE b* values. Lu et al. (2019) also reported that superchilled storage led to less change in the color of beef steak than in refrigerated samples. Accordingly, supercooling induced stability in the change color of pork loin samples at a similar level of freezing without phase transition. It was concluded that supercooling could maintain an acceptable quality for pork loins with higher storage temperatures compared to freezing for 16 days of storage.
The pH values of pork loin samples subjected to various treatments and storage periods are presented in Table 3. The pH of meat affects physicochemical properties such as WHC and tenderness (Kauffman et al., 1961). The pH of fresh pork loin sample was 5.64 and varied by treatment and storage periods. Overall, unfrozen meat had a lower pH than the frozen samples. After eight days of storage, the pH values for refrigerated and supercooled pork loin samples were approximately 5.6, whereas those of frozen meats were 5.68 and 5.87, depending on the freezing temperature. After 16 days of storage, the pH values of the samples increased by approximately 0.1 units except for pork loin samples frozen at −18°C, which decreased to 5.78.
According to James (1972), the pH of fresh meat ranges from 5.5 to 5.8 and that of putrid meat is above 8.0. At slaughter, the pH of meat is approximately 7.2 and initially decreases due to the depletion of ATP and anaerobic glycolysis (Ko and Yang, 2001; Scherer et al., 2005). Numerous studies have demonstrated an increase in the pH of meat due to the production of basic substances including ammonia and amines by microbes and enzymes (Demeyer et al., 1979; Holley et al., 1994; Kim et al., 2004; Muela et al., 2010). Choi et al. (2002) also reported an increase in the pH of pork loins stored at 4°C, ranging from 5.61 to 5.81. Vacuum packaging prevented a significant pH shift in pork during refrigeration storage (Kim et al., 1998; Seong et al., 2009). Lu et al. (2019) also indicated that superchilling (storage at −4°C) limited the increase in the pH of meat within 12 weeks. Ding et al. (2020) reported slightly different trends in the pH of superchilled pork loins depending on the storage temperature. The pH of pork loins stored at −1°C gradually increased, whereas those of samples stored at −3°C and −2°C decreased and rebounded during 1 month of superchilling storage, ascribed to the disintegration of buffer proteins and the release of hydrogen ions resulting from formation of ice crystals.
In this study, the pH of supercooled pork loin sample was maintained during the 16 days of storage (p>0.05) and was comparable to that of the fresh state, which is consistent with the results obtained by Seong et al. (2009). This result may be attributed to the relatively air-tight packaging method used in this study. In contrast, the pH values of frozen samples significantly increased depending on the storage period (p<0.05). The results suggested that the supercooling treatment was helpful for retaining the original pH and related reactions.
The VBN values of pork loin samples subjected to various treatments and storage periods are presented in Table 3. The VBN of fresh pork loin samples was 5.84 mg/100 g, and that of pork loin samples stored by refrigeration was the highest among the treatment groups (p<0.05). After 8 days of storage, the VBN content of refrigerated pork loin sample was 6.67 mg/100 g. On the 16th day, the VBN level increased to 6.84 mg/100 g, although the difference was not significant (p>0.05). The VBN content also increased in the other samples but was not significantly higher than that of fresh meat (p>0.05). Regardless of the cooling treatment and storage period, all VBN values were approximately 6.10 mg/100 g.
VBN comprises ammonia and amines from disintegrated proteins in meat and is regarded as a freshness indicator for meat products. These nitrogen-containing substances are generated by the proteolytic activities of enzymes and bacteria (Ding et al., 2020; He et al., 2013). Thus, low temperature storage of meat is essential to reduce the amount of VBN by inhibiting the metabolic reactions associated with VBN compound generation (Choi et al., 1998; Lee and Jang, 2013). Ding et al. (2020) reported that superchilled pork loin stored at −3°C and −2°C had lower VBN than that stored at −1°C. Lower VBN content was also reported in superchilled Chinese yellow cattle than in refrigerated samples, though frozen cattle meat had the lowest VBN over 24 weeks (Lu et al., 2019). Superchilled grass carp fillet (Liu et al., 2013) and rabbit meat (Lan et al., 2016) had insignificant results for VBN. Therefore, supercooling treatment was superior in terms of VBN due to its lower value compared to refrigeration and no difference compared to freezing treatment, without undergoing freezing itself.
The TBARS values of pork loin samples subjected to various treatments and storage periods are presented in Table 3. The TBARS amount present in the fresh pork loin sample was 0.1927 mg MDA/kg, and all TBARS values were under 0.2500 mg MDA/kg. After 8 days of storage, the amount of TBARS in the refrigerated pork loin sample was 0.2380 mg MDA/kg and that in other samples was approximately 0.2100 mg MDA/kg, with no significant difference among treatments (p>0.05). After 16 days of storage, the TBARS values decreased, showing 0.1751–0.1898 mg MDA/kg depending on the treatment. Among the samples, the supercooled pork loin sample had the lowest TBARS content (p>0.05).
Lipid oxidation is a time- and temperature-dependent procedure associated with a rancid odor, discoloration, and carcinogenesis (Amaral et al., 2018; Domínguez et al., 2019). Thus, lipid oxidation of meat can be restrained by low temperature storage, similar to VBN. There have been studies on the effectiveness of refrigeration on lipid oxidation in pork and the results indicated that TBARS values increase with the longer storage periods (Choi et al., 1998; Kim et al., 2004; Seong et al., 2010). Superchilling was also performed to lower the TBARS of meats. Pomponio and Ruiz-Carrascal (2017) reported that superchilled pork loins had approximately 0.2 mg MDA/kg of TBARS after up to 12 weeks of storage. Ding et al. (2020) asserted that ice crystals formed by superchilling reduced TBARS in pork loins, and a lower storage temperature resulted in lower TBARS levels. Lu et al. (2019) observed a higher TBARS value in beefsteak than in frozen samples and ascribed these findings to damage in the cells during superchilling storage and leakage of prooxidants through the damaged regions.
In this study, all pork loin samples had trace amounts of TBARS, and a rancid odor induced by lipid oxidation was not detected after storage. It was concluded that the low-temperature treatment of pork loin samples limited lipid oxidation. Furthermore, the initially low lipid content of the pork loin samples could be the reason for the low TBARS values throughout the storage period. Consequently, low-temperature treatment is effective for restricting lipid oxidation, and supercooling storage results in lipid oxidation stability in pork loin.
The TAC for pork loin samples subjected to various treatments and storage periods is presented in Table 3. The TAC of the fresh pork loin sample was 3.8 Log CFU/g, similar to the value obtained by Choi et al. (1998), who reported that fresh pork loin had 2–4 Log CFU/g of TAC. After 16 days of storage, the TAC of refrigerated pork loin sample was 6.1 Log CFU/g, which was the highest value (p>0.05). Refrigeration induced a large amount of microbial growth in pork loin samples due to the higher storage temperature and the TAC exceeded 6 Log CFU/g, which meets the criteria for putridity (Egan and Shay, 1982). The TAC for both supercooled and frozen pork loin samples at −18°C was 3.3 Log CFU/g and that of samples frozen at −3°C was 3.6 Log CFU/g, showing a considerable reduction compared to refrigerated samples.
Microbial activity is a primary factor related to the shelf life of meat and is affected by temperature (Hong et al., 2012; Lu et al., 2019). Microorganisms decompose macromolecules such as proteins into smaller substances, including ammonia, amines, mercaptan, and hydrogen sulfide, which are the origins of the off-odor (Choi et al., 2002; Nychas and Arkoudelos, 1990; Ordonez et al., 1991). Microbial spoilage also results in discoloration and/or slime production (Reid et al., 2017). Thus, a low storage temperature is indispensable for controlling microbial counts in meat (Ye et al., 2020). Bellés et al. (2017) also reported that superchilled (−1°C) lamb had a lower TAC than samples refrigerated at 4°C. Ye et al. (2020) recommended storing pork at −3°C to inhibit microbial growth.
In this study, the overall pattern of TAC depending on treatment was similar to the trend for VBN. Refrigeration induced a large amount of microbial growth in pork loins due to the higher storage temperature and regarded to be rotten and inedible (Egan and Shay, 1982; Nottingham, 1982). Concomitant with this criterion, refrigerated pork loins had strong off-odors at the end point of storage. Freezing was effective to inhibit the microbial spoilage of pork loins during the storage period. Compared to other treatments, supercooling also prevented an increase in the TAC of pork loins, with similar TAC values as control and frozen samples without freezing. Consequently, it was concluded that supercooling can be a profitable storage treatment method for pork loins without phase transition, as it suppresses microbial spoilage during 16 days of storage.
Conclusion
A stepwise algorithm was designed to achieve supercooling storage of pork loins. Using this algorithm, pork loin samples successfully remained in a supercooled state for 16 days, and the meat quality was maintained. Supercooled pork loin samples showed superior drip loss and similar levels of cooking loss compared to the other treatment groups. Unfrozen pork loin samples had a higher WHC than frozen samples, with no marked change compared to fresh meat. Supercooled samples had pH values similar to those of fresh meat, whereas frozen samples showed a significant increase. The shear force for refrigerated and supercooled meats decreased during the initial storage stage and remained constant until the end of storage. Supercooling treatment also inhibited discoloration during the storage period, resulting in total color difference values similar to those of frozen samples without freezing. The pattern for VBN is analogous to that of the total color. Importantly, supercooling treatment prevented microbial growth for up to 16 days. In contrast, although refrigerated pork loin samples had a higher WHC, they presented discoloration and the highest VBN values. These samples exceeded the criteria for putridity and were regarded as inedible. Freezing generally maintained meat quality and prevented an increase in the TAC. However, freezing and thawing reduced the WHC of pork loin samples. This may be attributed to mechanical damage to the membranes from the ice crystals formed during the phase transition. Consequently, supercooling storage was effective to maintain the freshness of pork loins for up to 16 days.