Introduction
The trend of a rapidly aging society has attracted significant attention. By 2050, nearly two billion will be over 60 years old (United Nations, 2019). Therefore, strategies to deal with aging-related issues to support a good quality of life have become crucial. Food intake and dietary patterns are pivotal factors for healthy aging. Nutrition plays an important role in controlling age-related physiological changes and chronic diseases; inadequate nutritional consumption negatively affects health and impairs important functional abilities (Calligaris et al., 2022). Protein intake, which has a direct effect on muscle anabolism, is particularly important in the elderly population to prevent sarcopenia, which leads to frailty and disability due to the considerable decline in muscle mass (Lee et al., 2021a). However, all physical and physiological processes are greatly affected by aging, and in the elderly, unlike with young adults, protein digestion can be inhibited due to poor oral health and the deterioration of their gastrointestinal function (Hernández-Olivas et al., 2020). In this context, the selection of a dietary protein source tailored to the specific health status of the elderly can prevent malnutrition by maintaining a nutritionally balanced diet and reducing several health problems.
Poultry meat, especially chickens with high protein content, has attracted attention due to consumer concerns about cardiovascular diseases caused by the overconsumption of red meat (Shin and Choi, 2022; Zou et al., 2018b). The low caloric property of chicken meat due to its lower fat content compared to other types of meat also appeals to consumers for a healthier diet (Barido et al., 2021; Kralik et al., 2018; Park et al., 2022). Moreover, a study by Hernández-Olivas et al. (2022) suggested that chicken meat is an ideal choice for the elderly among four different types of meat (beef entrecote, pork loin, turkey breast, and chicken breast) in terms of protein digestibility. Among the different chicken cuts, breasts and thighs have a significant preference and popularity among consumers (Haley, 2001). These two cuts consist of a distinct composition of muscle fibers that represent characteristics of slow- and fast-twitch muscle fibers, depending on their muscle contraction speed and respiration activity (Xiong, 1994). Myosin, a representative protein with different isoforms depending on the muscle fiber types, participates in muscle contraction with other myofibrillar proteins, such as tropomyosin and troponin, and is generally considered as the molecular marker of fiber types (Choi and Kim, 2009). Myosin is composed of two myosin heavy chains (MHC) and four myosin light chains that regulate myosin motor activity. Among the several types of myosin isoforms, four of them (including I, IIA, IIX, and IIB) are the predominant MHC isoforms in skeletal muscle. MHC I and the other three isoforms are expressed in slow-twitch (oxidative or type I) and fast-twitch (glycolytic or type II) muscle fibers, respectively (Pette and Staron, 2000) and the avian skeletal muscle is primarily composed of these two types of muscle fibers.
Although the content of each muscle fiber varies depending on experimental method, breed, age, and study, a higher slow-twitch muscle fiber content in leg muscles, including the thigh, has been consistently reported. Verdiglione and Cassandro (2013) suggested that the breast muscle of broilers comprises 100% fast-twitch fibers, whereas Du et al. (2017) observed 76%–79% slow-twitch fibers in broiler legs. Jaturasitha et al. (2008), who monitored the muscle composition of Thai native chickens, reported that the fiber count of type I was 1.1% and 18.2% of the total in the breast and thigh muscles, respectively. Moreover, type IIA (3.9% and 26.0% of the total in the breast and thigh, respectively) and IIB (95.0% and 55.8% of the total in the breast and thigh, respectively) also showed considerably different values. Apart from the differences in the fiber types, in the case of chicken thigh, the presence of the various types of muscles might also contribute to the distribution of various types of proteins, unlike chicken breast.
As a result of this, we assumed that the thigh and breast have different digestive characteristics due to their metabolic, contractile, and enzymatic properties. Therefore, it is useful to monitor their biochemical properties to understand the differences in the protein bioavailability of the two muscles. Although several studies have compared the in vitro protein digestibility between meat sources, research on the digestive behavior of proteins in different muscle fiber types is limited. Zou et al. (2018a) reported that the different muscle fiber types can be attributed to the different compositions of muscle proteins and their digestive susceptibility. Moreover, since the muscle fibers have different resistance to thermal denaturation (Vaskoska et al., 2021), we hypothesized that chicken breast and thigh may have different digestive behavior especially after cooking. Therefore, this study aims to investigate the structural and functional properties of chicken breast and thigh muscle. In addition, we evaluate the in vitro protein digestibility of the two muscles in an elderly digestion model to determine the muscle with ideal protein source.
Materials and Methods
Five commercially processed broiler carcasses were purchased from a local market (Daejeon, Korea). The breast fillets and thighs (mainly composed of iliofibularis and iliotibialis) were removed from the carcasses along with excessive fat and connective tissues, followed by grinding (CH580, Kenwood, Havant, UK) for the experimental analysis. Each ground muscle was vacuum-packed and stored at −70°C after sampling until analysis.
The pH of the muscles was monitored immediately after sample collection. An aliquot (9 mL) of distilled water was added to 1 g of muscle, followed by homogenization (T25 basic, IKA-Werke, Staufen, Germany) for 30 s. The homogenate was centrifuged at 2,000×g (1580R, LaboGene, Lillerød, Denmark) and the supernatant was filtered through filter paper (No. 4, Whatman, Maidstone, UK). The pH of the filtrate was measured using a pH meter (SevenEasy, Mettler-Toledo, Schwerzenbach, Switzerland).
The proximate composition including moisture, crude protein, crude fat, and crude ash content of the chicken muscles was monitored according to AOAC methods 950.46, 928.08, 960.39, and 923.03, respectively.
A 10% trichloroacetic acid (TCA)-soluble fraction was obtained according to the method described by Lee et al. (2020a). The α-amino group content in the TCA-soluble fraction and digesta was monitored through reaction with o-phthaldialdehyde reagent (Church et al., 1983). The absorbance of the mixture was measured at 340 nm using a plate reader (Varioskan LUX, Thermo Fisher Scientific, Waltham, MA, USA). A standard curve was produced with glycine, and the crude protein content was measured using the Kjeldahl method (AOAC method 928.08).
Protein carbonyl content was monitored to evaluate protein oxidation, following the method described by Estévez (2011). Chicken muscle (1 g) was homogenized with 10 mL of 0.6 M NaCl in 20 mM sodium phosphate (pH 6.5) at 13,000 rpm for 30 sec (T25 basic). The mixture was used as the sample to monitor protein carbonyl content. The samples were reacted with dinitrophenylhydrazine and the absorbance of the mixtures were read at 370 nm. Protein content was measured using a Bio-Rad protein assay (#5000006, Bio-Rad Laboratories, Richmond, CA, USA). Bovine serum albumin was used to create a standard curve and the absorbance was read at 595 nm. The protein carbonyl content was calculated using a molar absorptivity of 22,000 M–1 cm–1 and expressed in nmol carbonyl/mg protein.
Myofibrillar proteins were prepared according to the method of Liu and Xiong (1996). The chicken muscle (3 g) was homogenized with 25 mL of ice-cold buffer containing 0.1 M KCl, 2 mM MgCl2, and 1 mM ethylene glycol tetraacetic acid (T25 basic) at 13,000 rpm for 30 sec. The homogenate was centrifuged at 3,000×g for 10 min (1580R) to remove the supernatant and this procedure was repeated three times. Then, the pellet was homogenized with 20 mL of 0.1 M NaCl and centrifuged to remove the supernatant three times. The final pellet was suspended in a 0.1 M potassium phosphate buffer (pH 7.4). The protein content was measured using a Bio-Rad protein assay.
The myofibrillar protein extracts were diluted to a concentration of 2 mg/mL for SDS-PAGE analysis. The myofibrillar protein samples were reacted with a sample buffer (EBA-1051, Elpis Biotech, Daejeon, Korea) at a ratio of 1:1 (vol/vol) at 90°C for 10 min. Electrophoretic separation was conducted using 12.5% polyacrylamide gel in an electrophoresis system (AE-6531 mPAGE, ATTO, Tokyo, Japan) and a protein ladder (3454A, Takara Bio, Shiga, Japan) was loaded to figure out the molecular weights of protein bands. The protein bands were stained using a solution containing Coomassie brilliant blue and de-stained using a solution containing 10% acetic acid and 30% methanol. The SDS-PAGE gel was scanned using Epson Perfection V850 Pro (V850 Pro, Epson, Long Beach, CA, USA).
Myosin was extracted using the method previously reported by Reddish et al. (2005) with some modification. The chicken muscle (1 g) was solubilized in buffer solution with high content of salt [0.04 M sodium pyrophosphate, 1 mM MgCl2, and 2 mM ethylene diamine tetraacetic acid (EDTA), pH 9.5] and then precipitated by the buffer solution with low content of salt (0.02 M KCl, 2 mM KH2PO4, and 1 mM EDTA, pH 6.8). The final pellets were suspended in 0.6 M KCl in a 20 mM potassium phosphate buffer (pH 7.0), and the protein content was measured using a Qubit Protein Assay Kit (A50669, Thermo Fisher Scientific).
The intrinsic tryptophan fluorescence intensity of myosin extract (protein concentration of 0.5 mg/mL) was observed using the method reported by Lee et al. (2021c). Scanning of the myosin extract was conducted between 300 and 400 nm at an excitation wavelength of 280 nm (Varioskan LUX), with a scanning speed of 1,000 nm/min, and excitation and emission slit widths of 5 nm.
The secondary structure of the myosin extract was measured using circular dichroism spectroscopy (Chirascan VX, Applied Photophysics, Leatherhead, UK). The myosin extract was diluted to a protein concentration of 0.5 mg/mL. A single spectrum was obtained with two replicates in a scan rate of 100 nm/min, a response time of 0.25 s, and a bandwidth of 1.0 mm. The data were expressed in millidegrees, and the scanning range was set between 200 and 260 nm. To estimate the content of secondary structural components, including α-helices, β-sheets, β-turns, and random coils in the spectrum, CDNN software (version 4.0, Gerald Böhm, Bioinformatics, Germany, CD Spectra Deconvolution Ver. 2.1) was used.
For in vitro digestion, the ground chicken muscles were vacuum-packed and cooked at 80°C to reach a core temperature of 75°C, followed by cooling to 25°C. Cooked samples were chopped to simulate mastication.
The elderly in vitro digestion model herein was designed based on previous studies by Hernández-Olivas et al. (2020) and Minekus et al. (2014). All the digestive enzymes that were used herein were purchased from Sigma-Aldrich (St. Louis, MO, USA). The simulated salivary fluid (pH 7.0), gastric fluid (pH 6.0), and duodenal fluid (pH 7.0) contained 75 U/mL α-amylase from Aspergillus oryzae (EC 3.2.1.1), 1,500 U/mL pepsin from Porcine mucosa (EC 3.4.23.1), 50 U/mL trypsin (EC 3.4.21.4) and 12.5 U/mL chymotrypsin (EC 3.4.21.1) from bovine pancreas, 1,000 U/mL pancreatic lipase from porcine pancreas (EC 3.1.1.3), and 5 mM porcine bile extract (EC 232-369-0). Digestive fluid was mixed with the digesta from the previous compartment at 50:50 (vol/vol) during digestion. Each digestion was conducted for 120 min at 37°C and a rotational speed of 100 rpm, except for oral digestion, which was conducted for 2 min. All digesta samples were stored at −70°C until analysis, immediately after digestion. Control samples were prepared for digestion under the same conditions through addition of distilled water instead of meat samples to exclude protein content from the digestive enzymes.
Herein, the size fractionation of the digesta was conducted to determine protein digestibility after in vitro digestion. After sequential filtration using a centrifugal filter with molecular weight cut-offs of 10 and 3 kDa (Amicon Ultra-15, Millipore, Billerica, MA, USA) according to the manufacturer’s protocol, the protein content of the filtrate and whole digesta was measured using the Kjeldahl method to represent the amount of protein digested under 3 kDa. Protein digestibility was calculated using the following Eq. (1):
This study used three iterations in three batches with analysis using a mixed model, and the batches (carcasses) were described as random effects. The least-squares mean and SE of the least-squares mean was used to express the results. The significance of the main effects was evaluated using Tukey’s multiple comparison test (p<0.05). The results were statistically analyzed using SAS software (version 9.3, SAS Institute, Cary, NC, USA).
Results and Discussion
Table 1 shows the results of the muscle characterization in this study. The pH of chicken breast and thigh used in this study was 5.81 and 6.49, respectively, with significantly higher pH in the thigh (p<0.05). The breast and thigh showed different proximate compositions, with higher crude protein and crude ash contents in the breast (p<0.05), and conversely, higher crude fat content in the thigh (p<0.05). Moreover, the thigh had higher protein carbonyl content than the breast (p<0.05).
Muscle fiber type differentiates the contractile characteristics and metabolic patterns (glycolytic and oxidative) that influence muscle-to-meat conversion and overall meat quality. As the slow- and fast-twitch muscle fibers have oxidative and glycolytic metabolism before slaughter, respectively, muscles predominantly composed of fast-twitch fibers possess more glycogen than those composed of slow-twitch fibers (Karlsson et al., 1999). The lower glycogen content in type I fibers can inhibit pH decline in muscles that are rich in type I fibers after slaughter (Vaskoska et al., 2021), which explains the higher pH of the thigh. Moreover, compared to fast-twitch fibers, slow-twitch fibers have a greater ability to use cellular lipids as fuel for ATP production due to their high mitochondria content and respiratory enzymes, resulting in high triglyceride content in muscle fibers (Karlsson et al., 1999). Therefore, the higher stored triglyceride content in the thigh resulted in higher crude fat and lower crude protein content than in the breast. This oxidative metabolic property of slow-twitch fibers also requires a greater ability to deliver oxygen to the muscle; as such, the chicken thigh has a higher heme protein content (including hemoglobin and myoglobin) than the breast (Gong et al., 2010). As heme proteins and transition metal ions are important factors that affect the oxidative susceptibility of meat proteins (Jongberg et al., 2014), the metal ion-induced acceleration of protein oxidation results in higher protein carbonyl content in the thigh.
Additionally, the α-amino group content in the 10% TCA-soluble fraction that contained small amino acids and peptides with 3–4 residues was higher in the thigh (p<0.05), indicating greater postmortem protein degradation. In general, fast-twitch fibers are considered to have faster postmortem proteolysis due to having a higher calpain-to-calpastatin ratio than slow-twitch fibers, as calpastatin activity is positively correlated with slow MHC isoforms (Christensen et al., 2004). However, calpain is highly sensitive to pH and exhibits optimum activity at neutral pH (Bhat et al., 2018). The rapid pH decline and lower ultimate pH of the breast may have induced lower calpain activity and an increase in protein denaturation, resulting in a greater breakdown of proteins in the thigh. However, Christensen et al. (2004) reported that the postmortem degradation of proteins depends more on the variations between muscles rather than the fiber type itself, and that the difference in the α-amino group content cannot be entirely explained by muscle fiber composition.
Overall, the two muscles exhibit different biochemical traits, which may be attributed to differences in their metabolic processes.
The previous section confirmed that chicken breast and thigh muscles have different muscle properties. To understand the difference in the digestive susceptibility of the two muscles, their structural characteristics and biochemical traits should be determined, because protein structure is an important factor that determines the accessibility of digestive enzymes to their cleavage sites in meat proteins (Lee et al., 2021a). Therefore, we will discuss the structural differences in the myofibrillar proteins, which are the major proteins in the muscle, between the two muscles.
The SDS-PAGE electrophoretogram of myofibrillar proteins showed different protein bands between the breast and thigh (Fig. 1A). The most noticeable difference was observed in the tropomyosin α- and β-chains. Although the tropomyosin β-chain appeared in both lanes, there was no tropomyosin α-chain in the thigh myofibrillar protein lane. In myofibrillar proteins, components such as troponin or actin exist as a single isoform, whereas others such as myosin and tropomyosin exist in several isoforms, which may differ depending on the muscle fiber type (Schevzov et al., 2011). According to a study by Heeley et al. (1985), although the α- and β-subunits of tropomyosin are the predominant components in both slow- and fast-twitch muscle fibers at birth, differentiation into slow-twitch fibers is accompanied by a decline in α-tropomyosin content. Therefore, the absence of the tropomyosin α-chain band in the thigh can be explained by the higher slow-twitch muscle fiber content compared to the breast. Moreover, the myosin light chain-3 band was weaker in the breast than in the thigh (Fig. 1A). Stuart et al. (2016) reported that although myosin light chain-1 is predominant in all fiber types, myosin light chain-3 is almost non-existent in fast-twitch muscle fibers. This result suggests that myosin light chain-3 is an essential phenotype of type I muscle fibers.
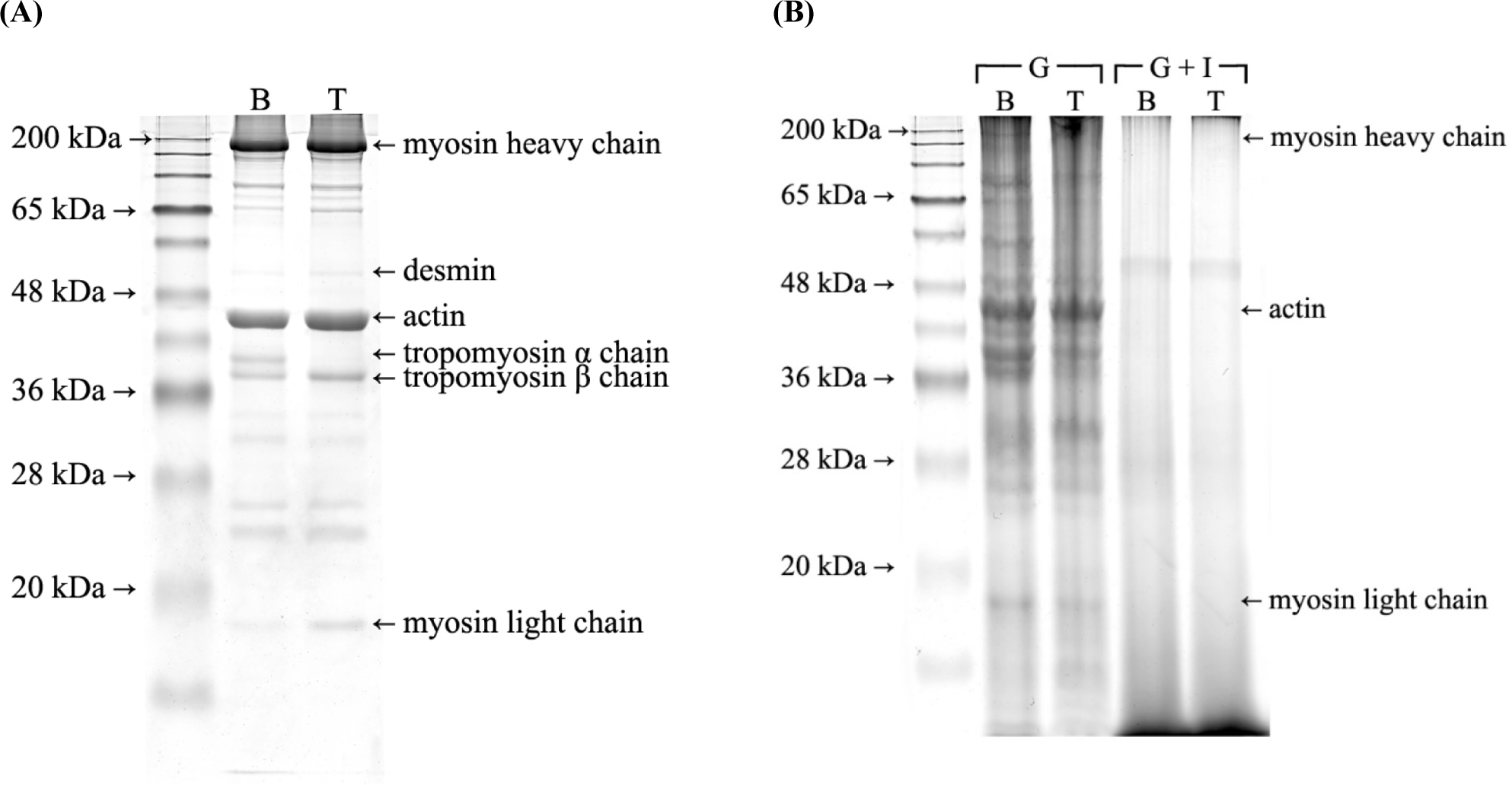
The hydrophobicity of proteins is known to largely contribute to their structural stability. The intrinsic fluorescence intensity of tryptophan, an aromatic amino acid, was monitored to determine the aromatic hydrophobicity of myosin in chicken breast and thigh tissues (Fig. 2). In the wavelength range of 300 to 400 nm, the breast muscle had a higher intrinsic tryptophan fluorescence intensity than the thigh muscle (Fig. 2A). When comparing the fluorescence intensity at 328 nm, which showed the highest intensity in both muscles (Fig. 2B), the breast had a significantly higher fluorescence intensity (p<0.05). This result indicates that the thigh has a higher aromatic surface hydrophobicity than the breast, which is consistent with previous studies. Boyer et al. (1996) reported that both aromatic and aliphatic hydrophobicity was higher in slow-twitch myosin than in fast-twitch myosin; the study showed that the aromatic surface hydrophobicity of slow-twitch myosin was 1.5-fold higher than that of fast-twitch myosin. Glorieux et al. (2017) also reported higher aromatic surface hydrophobicity in the thigh due to different myosin isoforms in the two muscles. Therefore, it appears that slow-twitch myosin has more hydrophobic residues on its surface than fast-twitch myosin. As the hydrophobic residues exposed on the surface form aggregates with hydrophobic interactions by thermal treatment (Mitra et al., 2017), the higher surface hydrophobicity of the chicken thigh can negatively influence the digestive susceptibility of proteins. This is further discussed in the succeeding sections.
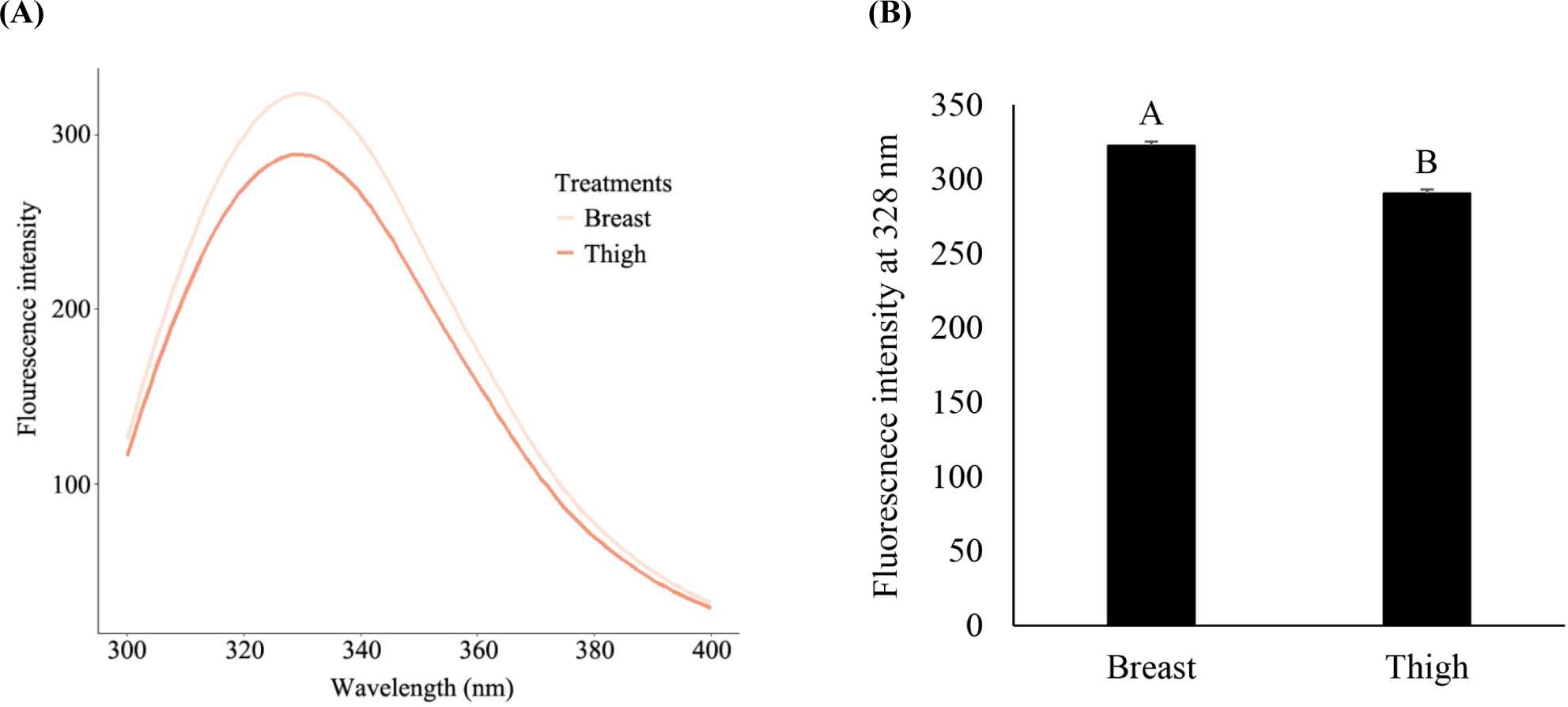
The relative percentages of the secondary structural components of myosin in the chicken breast and thigh are presented in Table 2. Myosin in the thigh had higher α-helix content and lower β-sheet content than that in the breast (p<0.05). There was no significant difference in the β-turn and random coil composition between the two muscles (p>0.05). Although few studies have compared and discussed the secondary structure of myosin between muscle fiber types, muscles primarily consisting of slow-twitch fibers were reported to have a higher α-helical structure than muscles with a relatively high fast-twitch fiber content (Bozkurt et al., 2010; Katemala et al., 2021). This difference in myosin structure may have been caused by the different isoforms. Though both α-helices and β-sheets are ordered structures, β-sheets generally represent a more compact and aggregated structure with intramolecular hydrogen bonding (Tan et al., 2021). Moreover, as α-helices are often positively correlated with protein digestibility (Bai et al., 2016; Han et al., 2019), this difference in the secondary structure of myosin between the two muscles can also contribute to digestive characteristics.
Treatment | α-Helix | β-Sheet | β-Turn | Random coil |
---|---|---|---|---|
Breast | 13.87B | 27.20A | 17.44 | 41.50 |
Thigh | 14.92A | 26.35B | 17.54 | 41.19 |
SEM1) | 0.121 | 0.208 | 0.055 | 0.191 |
The in vitro digestion in the elderly digestion model was divided into two compartments for gastric and gastrointestinal (adding simulated duodenal fluids following gastric digestion) digestion, as meat proteins are reported to be digested in different stages of digestion (Lee et al., 2021a). We employed three experimental parameters in the digesta to determine differences in the digestive characteristics of the two muscles. The α-amino group content was used to measure the release of small amino acids and peptides during in vitro digestion (Fig. 3A). In the SDS-PAGE electrophoretogram of the gastric and gastrointestinal digesta, the protein that can be preferentially digested is estimated by the disappearance of protein bands (Fig. 1B). As protein bioavailability is much more important in the elderly for protein anabolism and prevention of sarcopenia (Lee et al., 2021b), we also monitored the protein content digested below 3 kDa (Fig. 3B). A previous study reported that proteins with a molecular weight less than 3 kDa can be absorbed in the small intestine (Vlahou et al., 2018); therefore, they can be used to evaluate the amount of protein absorbed after digestion.
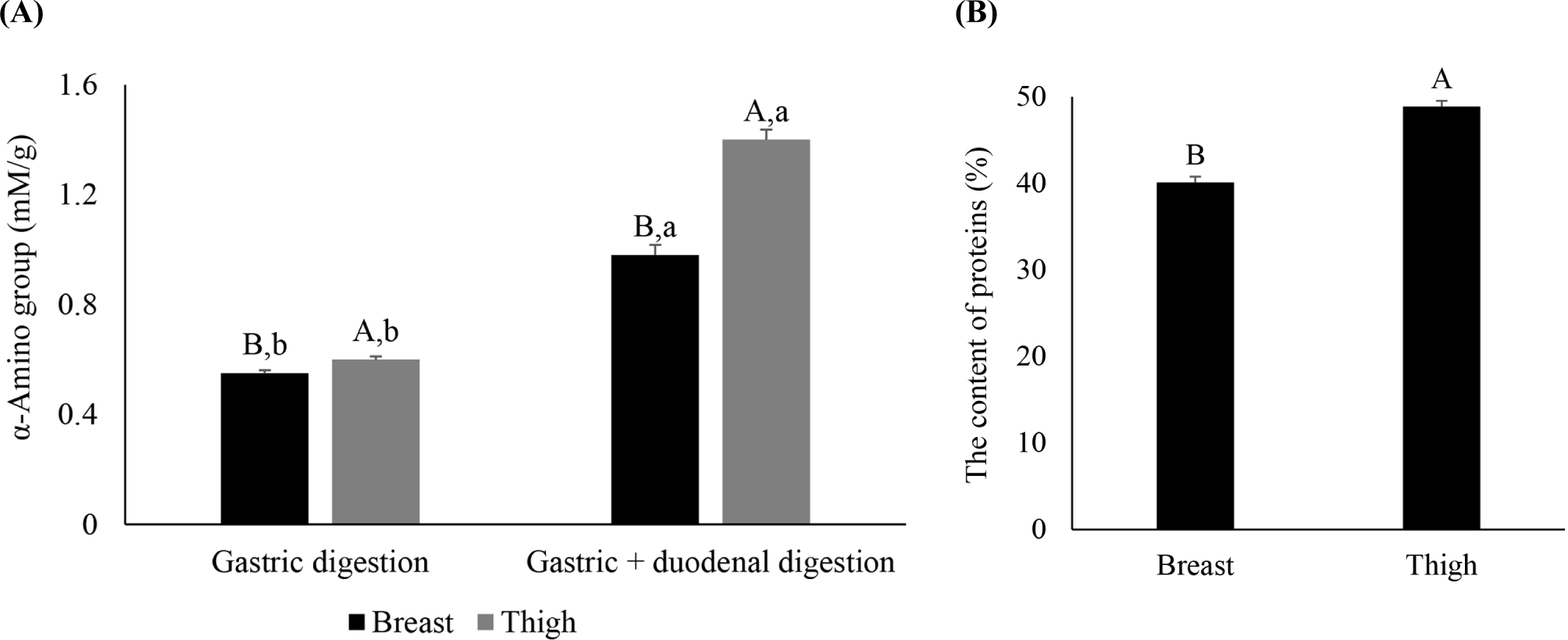
The thigh had higher α-amino group content than the breast after gastric and gastrointestinal digestion (Fig. 3A, p<0.05), and the difference between the two muscles increased after gastrointestinal digestion. The protein content digested below 3 kDa after gastrointestinal digestion was also higher in the thigh than in the breast (Fig. 3B, p<0.05). Therefore, in the elderly digestion model, the thigh had higher in vitro protein digestibility than the breast.
The higher in vitro protein digestibility in the thigh can be explained by the difference in thermal stability between the two muscles, as we cooked the chicken muscles at 80°C before in vitro digestion because meat is generally cooked before consumption. Following this, we discovered higher intrinsic tryptophan fluorescence intensity in the breast than in the thigh, indicating higher aromatic surface hydrophobicity of the thigh. In general, exposed hydrophobic residues form aggregates via hydrophobic interactions during thermal treatment (Mitra et al., 2017), potentially resulting in a decrease in protease accessibility (Lee et al., 2021a). However, according to the results of Boyer et al. (1996), although the surface hydrophobicity of slow-twitch myosin before heating is higher, fast-twitch myosin shows higher hydrophobicity after thermal treatment, indicating higher susceptibility of the hydrophobic residues in fast-twitch myosin to the heat-induced protein unfolding. This explanation is consistent with the results of Vaskoska et al. (2021), who compared the thermal stability between muscles primarily comprised fast- and slow-twitch muscles; the study reported that fast-twitch muscle fibers have lower thermal stability with an earlier onset of thermal denaturation and a greater extent of aggregated strands after cooking compared to slow-twitch fibers. Young et al. (1992) also reported a lower (by 10°C) heat-induced gelation temperature of myofibrillar proteins from fast-twitch fibers. This difference in thermal stability between the two fiber types may be related to their different amino acid compositions influencing the primary structure; for instance, slow-twitch myosin containing various substitutions in the light chain region with a shorter sequence of amino acids (Chikuni et al., 2004). Moreover, the lower ultimate pH of the breast may have also affected the lower thermal stability, as Böcker et al. (2006) reported that more aggregated strands were observed in muscles with lower pH. We also observed the secondary structure in Table 2, wherein the breast had lower α-helix content and higher β-sheet content than the thigh. Thus, the breast may have a more compact structure and a lower protease accessibility, even before cooking. As heat-induced protein aggregation can hinder the action of digestive enzymes via protein aggregation followed by the burial of their cleavage sites (Lee et al., 2021a), this may have resulted in lower protein digestibility in the breast than in the thigh.
Therefore, aggregation-induced steric hindrance of myofibrillar proteins and digestive enzymes may have been greater in the breast. In the SDS-PAGE electrophoretogram of the digesta (Fig. 1B), although there were no noticeable differences in the protein bands of the gastrointestinal digesta, there were more bands in the gastric digesta of the breast than that in the thigh. In the gastric digesta, the actin band appeared with a relatively higher intensity than any other protein, indicating the digestive resistance of actin in the gastric phase, as we have already observed in our previous studies (Lee et al., 2020b; Lee et al., 2021c). In particular, the breast still had troponin and tropomyosin α- and β-chains though they disappeared in the thigh. Troponin and tropomyosin are associated with actin filaments by stabilizing the filament and controlling the access of actin-binding proteins (Schevzov et al., 2011). Therefore, the degradation of these two proteins can improve the digestive accessibility of myofibrillar filaments by reducing their structural integrity and steric hindrance (Lee et al., 2021a). However, as we have already discussed previously, the breast, which may have a more compact and aggregated structure than the thigh, appears to have lower digestive susceptibility to regulatory proteins, resulting in a lower overall digestion of myofibrillar proteins. The higher digestion of the thigh in the gastric phase may also have induced greater digestion in the intestinal phase due to more disrupted and fragmented protein structure.
Aging is accompanied by the deterioration in the masticatory capacity and secretion of digestive juices that can impair protein digestion. The delay in digestion due to the elevated intragastric pH and deteriorated secretion of digestive enzymes contributes to slow gastric emptying in the elderly (Lee et al., 2021a). The impairment in protein digestibility reduces the absorbable amount of proteins so that the health problems such as sarcopenia and malnutrition occur. This is why the elders are advised to select the adequate amount and appropriate types of dietary proteins so that they can meet their protein requirements. Overall, the differences in the metabolic and contractile properties of the two muscles can be attributed to their different characteristics and structural properties. The chicken thigh, which was reported to have higher slow-twitch muscle fibers than the breast, showed faster and greater protein digestion in the elderly digestion model, resulting in higher in vitro protein digestibility. Therefore, the higher and faster digestion of chicken thigh than chicken breast can be a desirable property to improve overall digestive processes in the elderly. This result suggests that chicken thighs can be a better choice as a dietary protein source for the elderly due to their higher protein bioavailability. Although we have focused on the difference in myofibrillar proteins and the resulting in vitro protein digestibility of two muscles herein, different muscular compositions can also attribute to the different distribution of sarcoplasmic and stromal proteins. Therefore, future study is required to compare the overall distribution of meat proteins and resulting protein bioavailability in the muscles with different fiber compositions.
Conclusion
We aimed to compare in vitro protein digestibility between chicken breast and thigh muscles with different muscle properties in an elderly digestion model. As hypothesized, chicken breasts and thighs exhibit different biochemical and structural traits. In proximate composition, chicken thigh had higher crude fat and lower crude protein contents than chicken breast and protein carbonyl and 10% TCA-soluble α-amino group contents were higher in chicken thigh than in chicken breast. After in vitro digestion in the elderly digestion model, both the contents of the α-amino groups and the proteins digested under 3 kDa were higher in the thigh, indicating higher in vitro protein digestibility in the thigh than in the breast. Considering the absorption and availability of ingested proteins, the chicken thigh is therefore potentially a better choice than the chicken breast as a dietary protein source for the elderly due to its higher in vitro protein digestibility.