Introduction
Horse oil (HO) is extracted from the adipose tissue of horses and has long been used in various Asian countries owing to its anti-inflammatory, anti-bactericidal, and anti-pruritic properties. Off late, HO has gained increasing attention as an ingredient in cosmetic formulations because of its potential to restore the skin barrier and provide skin-moisturizing benefits (Park and Kim, 2021). HO can originate from different anatomical regions of the horse, including meat, bones, and abdominal fat. Notably, it contains elevated levels of unsaturated fatty acids (UFA) that closely resemble those found in the human skin, rendering it a desirable component for dermatological application (Maeng et al., 2018). Linoleic acid (C18:2n6, LA) and α-linolenic acid (C18:3n3, ALA) are the highest fatty acid among the polyunsaturated fatty acids (PUFA) in HO, known for their anti-inflammatory properties (Lee et al., 2018). LA and ALA (a precursor to omega-3 eicosapentaenoic acid and docosahexaenoic acid) may reduce the production and influence gene expressions of pro-inflammatory cytokines including tumor necrosis factor-alpha (TNF-α) and interleukin-6 (IL-6; Ren and Chung, 2007; Saiki et al., 2017). Also, they are important for maintaining skin homeostasis as they cannot be endogenously synthesized in the human body and must be acquired through external consumption (Simard et al., 2022). The anti-inflammatory activity of HO has already been reported by Kim et al. (2020) using UVB-induced photoaging and inflammation in hairless mice. Incorporating HO topically significantly inhibited IL-6 and TNF-α production induced by UV-B radiation. This feature underscores the potential therapeutic and cosmetic applications of HO in skin care.
The extraction process plays a crucial role in animal oil production and significantly influences its yield, quality, and chemical composition of the extracted oil. Traditional extraction methods that rely on heat and flammable solvents present environmental and human health hazards and impact the quality and bioactive constituents of the oil itself (Gaber et al., 2018). In contrast, supercritical fluid extraction has emerged as an environmentally sustainable technique that has been employed in diverse industries, including food, pharmaceuticals, and cosmetics, since the 1970s. This method uses solvents such as water, carbon dioxide, and ethanol, which are widely recognized as safe and operate at lower temperatures than commercial extraction processes. This low-temperature approach preserves the appearance and nutritional content of the extracted oils. Supercritical fluid extraction has been widely used to extract bioactive compounds from various plant-based matrices, including green tea (Kim et al., 2007), orange peel (Lee et al., 2001), ginseng (Woo et al., 2011), and leaves of Eurya japonica (Li et al., 2020). However, the use of supercritical fluid extraction to extract oil from animal-based sources remains has not been explored in detail.
Additional purification processes are required to improve the quality and eliminate undesired odors from the extracted oil. Deodorization represents the final phase of the oil refining process and is designed to eliminate any undesired off-odors and off-colors that may persist from the earlier stages (Hamm, 2003). The conventional approach for deodorizing animal oil involves conducting the process under vacuum conditions at a temperature of 180°C. This specific temperature regime is primarily employed to mitigate the risks of polymerization and geometrical isomerization during the purification process (Carvajal and Mozuraityte, 2016). An innovative approach to deodorization, diverging from its reliance on high temperatures, is the utilization of various deodorizing agents such as activated carbon (AC) and zeolite (Gibon et al., 2007). AC is renowned for its highly porous structure and substantial adsorptive surface area and has been used in oil purification processes. It can adsorb molecules from liquid and gas phases through physical and chemical adsorption mechanisms, including van der Waals forces (Castillejos et al., 2012). AC can be obtained from various sources, including cassava peels, rice husks, bamboo, and palm kernels. Palm kernels are a by-product of palm oil production and can be employed to produce palm activated carbon (PAC). PAC exhibits microporous properties like those of AC, endowing it with a significant surface area for the adsorption of undesired off-odors and off-colors in oil. Zeolite, characterized by their nanoporous structures and substantial surface areas, excel at adsorbing polar oxidation (Fuss et al., 2021). These materials, including AC, PAC, and zeolite, are widely used for catalysis, separation, and selective adsorption decontamination. Nevertheless, their effectiveness in purifying HO using the supercritical fluid extraction method, followed by deodorization, remains ambiguous and requires further exploration.
Therefore, the objective of this study was to elucidate the potential use of supercritical fluid extraction for HO extraction and investigate the efficacy of AC, PAC, and zeolite as deodorizers in HO purification.
Materials and Methods
Subcutaneous fat was obtained from the necks of 27-month-old Jeju horses from a local slaughterhouse on Jeju Island, Korea. The collected horse fat was cut into dimensions of 0.5×0.5 cm and subjected to supercritical fluid extraction following the method described by Li et al. (2020), with slight modifications. Subsequently, a four-step purification process was performed. The extracted horse fat was purified using a supercritical CO2 extraction system (ISA-SEFE0-500-0700-080 system, Ilshin Autoclave, Daejeon, Korea) at the Nano Bio Research Center in Naju, Korea. The primary extraction gas used was pure CO2 with C2H3OH as the co-solvent. The sample was exposed to a pressure of 400 bar and the extracted HO was collected at 400 bar and 40°C for 8 h. The extracted HO was then stored at –20°C for subsequent use.
The purification of HO from supercritical fluid extraction involves four stages: degumming, acid removal, washing and fractionation, bleaching and deodorization. In the degumming stage, 1% phosphoric acid (85%, Samchun Pure Chemical, Seoul, Korea) was added to the supercritical fluid extracted HO to remove the fat-soluble gums. Subsequently, 1% of 3N NaOH was added to remove free fatty acids, trace metals, and pigments during the acid removal process. The sample underwent two cycles of washing using distilled water, followed by stirring in a 50°C water bath with an automatic stirrer for 20 min to eliminate moisture. Separation was accomplished using a separation funnel during the washing and fractionation phases. In the final stage of bleaching and deodorization, various deodorants, including AC, PAC, and zeolite, were added at a concentration of 2% to remove the off-odor and undesirable color from the HO. The treatment groups were control group with no deodorant treatment (CON), AC-purified HO (ACHO), PAC-purified HO (PACHO), and zeolite-purified HO (ZHO). The deodorization process transpired in a shaking incubator for 30 min at a temperature of 50°C, and the ultimate purified HO from supercritical fluid extraction was obtained through vacuum filtration. Stringent safety protocols were adopted throughout each phase of the study.
The yields of HO purified from supercritical fluid extraction using various deodorant purification processes were analyzed and quantified using the equation established by Gudmundsson and Hafsteinsson (1997).
The malondialdehyde content in the samples was determined in accordance with the method outlined by Buege and Aust (1978). A total of 15 mL of distilled water was added to 5 g of sample and 0.05 μL of 7.2% butylated hydroxytoluene was then added. After thorough homogenization, 2 mL of 20 mM 2-Thiobarbituric acid (in 15% trichloroacetic acid) was added to 1 mL of the homogenate mixture. The mixture was then kept in a 90°C water bath for 15 min. After cooling for 10 min, the sample was centrifuged at 2,000×g for 10 min. Thiobarbituric acid reactive substance (TBARS) was determined using a UV/VIS spectrophotometer (M2e, Molecular Devices, Sunnyvale, CA, USA) by measuring the absorbance at 531 nm with distilled water as the blank sample. The TBARS value was calculated by multiplying the absorbance value by a factor of 5.88.
Instrumental color analysis was conducted using a Chroma meter CR-400 colorimeter (Minolta, Osaka, Japan) to measure the CIE L*, CIE a*, and CIE b* of the samples. Each sample was subjected to ten measurements to ensure accuracy and reliability.
Fatty acid analysis was conducted by examining fatty acid methyl esters (FAME) in the samples using a gas chromatography (GC)/flame-ionization detection instrument (detailed operating conditions are listed in Table 1; Folch et al., 1957). Fat was extracted from the samples using a Folch solution (chloroform:methanol=2:1) and then transferred to a test tube. NaOH methanol solution (1.5 mL of 0.5 N) was subsequently added, followed by heating at 100°C for 5 min and cooling in a cold-water bath. The mixture was then homogenized with 2 mL of boron trifluoride-methanol solution (approximately 10%, Supelco, Bellefonte, PA, USA) to convert the fatty acids into methyl esters. After 2 min of heating at 100°C and another cooling phase, FAME was extracted by adding 2 mL iso-octane to the mixture. To complete the process, 1 mL of saturated NaOH was added and mixed thoroughly. Centrifugation was performed at 783 ×g for 3 min to facilitate the separation of the supernatant layer containing FAME, which was then transferred to a GC vial for subsequent analysis.
The profiles of volatile organic compounds (VOCs) in the samples were determined via headspace solid-phase microextraction (HS-SPME) analysis according to the method of Del Pulgar et al. (2013). The analysis comprises three key stages: 1) VOC extraction, 2) Gas chromatography-mass spectrometry (GC-MS) analysis, and 3) VOC flavor analysis. For VOC extraction, 5 g of the sample was introduced into a 20 mL glass vial sealed with septa and a crimp cap. Equilibration of VOCs in the headspace was initiated within a sealed vial and placed in a constant-temperature water bath (40°C) for 20 min. An SPME fiber (50/30 μm DVB/Carboxen/PDMS, Supelco) was employed to absorb the VOCs by exposing the needle on the headspace, allowing for a 30 min absorption period.
The VOCs absorbed onto the SPME fibers were analyzed by GC-MS (Agilent 8890 GC/5977B MSD, Agilent Technologies, Palo Alto, CA, USA). Helium served as the mobile phase, and a DB-5MS analytical column (30 m×0.25 mm id, 0.25 μm) was utilized at a flow rate of 1.3 mL/min. The inlet temperature was set at 250°C, the detector temperature was 280°C, and the oven was initially held at 40°C for 10 min in gradient mode, increased to 250°C at a rate of 5°C/min, and then held for 5 min. The fragrance component absorbed onto the SPME fiber was desorbed by exposure to an injector for 15 min.
The VOC flavor within the samples, as derived from the GC-MS results, was identified using the linear retention index (LRI) and mass spectral library data (NIST21 mass spectral library, National Institute of Standards and Technology, Gaithersburg, MD, USA), along with alkane standard materials (C10–C26). The VOC components were expressed in units of 1×106 of the area (A.U. ×106). Additionally, the flavor characteristics of each VOC were analyzed in accordance with Magagna et al. (2016) using FlavorDB (https://cosylab.iiitd.edu.in/flavordb/), FooDB (www.foodb.ca/), and Flavornet (http://www.flavornet.org).
Statistical analysis of the data (n=3) was performed using a one-way analysis of variance, followed by Tukey’s range test as the post-hoc test. Statistical significance was set at p<0.05. SAS 9.4 (SAS Institute, Cary, NC, USA) was used for the analysis, and superscripts indicated significant differences among treatments. The identified VOC compounds were subjected to additional partial least squares-discriminant analysis (PLS-DA) and heatmap analysis using MetaboAnalyst 5.0 online analysis software.
Results and Discussion
The TBARS assay is a rapid and convenient method to determine the levels of oxidative products derived from fatty acids in animal products (Oancea et al., 2022). In agreement with the yield percentages, the TBARS values of CON remained consistent throughout the purification process involving various deodorants (Table 2). Interestingly, the TBARS value of CON in this study was found to be below the established standard TBARS value of oil designated for human consumption, which is typically within the range of 7–8 mg MDA/kg of oil (Monte et al., 2015). Deodorants are frequently used to remove impurities from oils, often producing transparent final products. Additionally, deodorants such as AC, PAC, and zeolite possess catalytic properties that expedite the decomposition of oxidizing compounds during oil purification (Hamm, 2003). These versatile substances have applications in various industries including deodorization, purification, decolorization, and catalysis. Functioning as deodorizers, they efficiently eliminate unwanted volatile off-odors through van der Waals forces while adsorbing particles responsible for undesirable coloration on their microporous surfaces during purification (Castillejos et al., 2012).
a–d Mean values within the same rows with the different superscripts are significantly different among treatments (p<0.05).
TBARS, thiobarbituric acid reactive substance; CON, supercritical fluid extracted HO without deodorant; ACHO, supercritical fluid extracted HO purified with activated carbon; PACHO, supercritical fluid extracted HO purified with palm activated carbon; ZHO, supercritical fluid extracted HO purified with zeolite; HO, horse oil.
The use of supercritical fluid extraction with various deodorizing agents during the purification process yielded substantial alterations in the color attributes of CON. Color is a pivotal quality parameter of vegetable and animal oils. The CON exhibited lower CIE L* and CIE a* values (54.52–56.02 and 0.14–0.24, respectively) compared to foal perirenal fat, which recorded a CIE L* and CIE a* value of 68.2 and 2.4, respectively (Álvarez et al., 2015). Similarly, in a comparative analysis of fat color among Jeju horses, Halla horses, lard, and beef-tallow, Park et al. (2019) reported that the liquid state of fat from Jeju and Halla horse meat, when subjected to temperatures below 60°C, yielded higher CIE L* values and lower CIE a* and CIE b* values, in contrast to CON and HO with deodorants in this study. The color profile of animal fat is an important quality indicator for both producers and consumers. In this regard, the CIE L*, CIE a*, and CIE b* values have been widely used to evaluate animal fat color. Notably, the CIE b* serves as a prominent factor in evaluating the color of animal fats. It has been found that animal fat with lower CIE b* values is often preferred by the majority of consumers due to its favorable sensory attributes (Ardeshiri and Rose, 2018). It is crucial to acknowledge that various factors, including feed intake and processing methods, can influence CIE b* values (Moloney et al., 2013).
The fatty acid compositions of the CON and HO with deodorants are shown in Table 3. CON and HO with deodorants exhibited higher concentrations of UFA than those of saturated fatty acids (SFA). CON and HO with deodorants had UFA concentrations between 60%–65% of all fatty acids present, as also observed previously (Maeng et al., 2018; Piao et al., 2019). The predominant UFAs found in CON and HO with deodorants were oleic acid (C18:1n9), LA (C18:2n6), and palmitoleic acid (C16:1n7). Oleic acid was found in CON and HO with deodorants with an average amount of 33.75%–33.81%, aligning with previous studies (Kim et al., 2020; Piao et al., 2019). Oleic acid has been reported to modulate inflammation and contribute to beneficial processes in skin tissue repair (Pereira et al., 2008). Although LA plays a vital role in maintaining the skin membrane fluidity, the levels observed in CON and HO with deodorants were slightly lower compared to those reported by Kim et al. (2020). Additionally, studies have found that palmitoleic acid in HO is associated with skin injury healing, and its proportion in HO exceeds that observed in lard and tallow (Park et al., 2019).
a,b Mean values within the same rows with the different superscripts are significantly different among treatments (p<0.05).
CON, supercritical fluid extracted HO without deodorant; ACHO, supercritical fluid extracted HO purified with activated carbon; PACHO, supercritical fluid extracted HO purified with palm activated carbon; ZHO, supercritical fluid extracted HO purified with zeolite; HO, horse oil; SFA, saturated fatty acid, UFA, unsaturated fatty acid, MUFA, monounsaturated fatty acid, PUFA, polyunsaturated fatty acid.
The SFA composition of CON and HO with deodorants ranged from 39.27% to 39.45%. Palmitic acid (C16:0) was the predominant SFA, followed by myristic acid (C14:0) and stearic acid (C16:0). This pattern is similar to findings that identified palmitic acid as the predominant SFA in HO, regardless of the extraction method employed (Piao et al., 2019). Notably, palmitic acid has been associated with reducing facial wrinkles and fine lines (Husein el Hadmed and Castillo, 2016). Overall, the results suggest that the supercritical fluid extraction method combined with various deodorants (AC, PAC, and zeolite) effectively purified HO without causing significant changes in the proportions of palmitoleic acid and LA.
Notably, this study found significant differences in the levels of ALA and eicosenoic acid (C20:1n9) in HO treated with deodorants (p<0.05). CON exhibited the lowest ALA content compared to HO with deodorants. The ALA contents in the CON, ACHO, PACHO, and ZHO treatments were 4.57%, 4.90%, 4.95%, and 4.99%, respectively. ALA, a PUFA, serves as a precursor to produce eicosapentaenoic acid (EPA) and docosahexaenoic acid. Its beneficial effects against various diseases have been also extensively studied, as evidenced by numerous scientific investigations (Yue et al., 2021). Additionally, ALA plays a crucial role in the formation of the skin barrier, and its anti-inflammatory properties contribute to skin health (Fan et al., 2020). Recent research conducted by Simard et al. (2022) underscored the significance of ALA in maintaining skin health, including its role in enhancing skin hydration and mitigating the risk of skin diseases. Additionally, the ratio of monounsaturated fatty acids (MUFA) to SFA showed no significant differences among the treatments, and the ratio of PUFA to SFA did not exhibit significant differences across the treatments. These findings indicate that the supercritical fluid extraction method combined with various deodorants (AC, PAC, and zeolite) did not significantly affect the overall ratio of MUFA and PUFA to SFA in purified HO.
Odor represents a critical quality parameter for HO. Oils derived from animal sources often have an unpleasant and distinctive odor. Given the subjectivity of sensory evaluation, which can vary among individuals (Moloney et al., 2013), it is imperative to analyze the odor of HO through chemical analyses, such as the identification of VOCs. The VOCs in the CON and HO with deodorants are listed in Table 4. The VOCs were classified into seven groups: alcohols, aldehydes, esters, furans, hydrocarbons, ketones, and other unclassified compounds, with 127 identified compounds. Among these, the hydrocarbons group emerged as the most prominent category among the other VOC groups across all treatments. In this study, the presence of undesirable odors in HO may be attributed to compounds such as 1-octen-3-ol and nonanal. While we cannot state that these compounds are solely responsible for the undesirable odor in HO, their detection in our study aligns with findings from horse meat in previous studies (Beldarrain et al., 2022; Maggiolino et al., 2019; Sujiwo et al., 2024; Tateo et al., 2020). These VOCs were also present among VOCs detected in other meat sources (Bleicher et al., 2022) and salami (Moretti et al., 2004). According to Maggiolino et al. (2019), nonanal is characterized by grassy, tea, vegetable, lemony, sour, beefy odor profile. VOC such as nonanal, heptanal, octanal, decanal, (E)-2-decenal and (E)-2-undecenal are relevant contributors of cooked horse meat aroma have been reported as major oxidation products derived from oleic acid (Beldarrain et al., 2022). Furthermore, 1-octen-3-ol has been recognized as a significant contributor to the overall flavor profile of boiled pork due to its higher odor activity values (Vilar et al., 2022).
1) Flavor profile of horse oil volatile organic compounds analyzed using FlavorDB (https://cosylab.iiitd.edu.in/flavordb/), FooDB (www.foodb.ca/), and Flavornet (http://www.flavornet.org).
a–c Mean values within the same rows with the different superscripts are significantly different among treatments (p<0.05).
LRI, linear retention index; CON, supercritical fluid extracted HO without deodorant; ACHO, supercritical fluid extracted HO purified with activated carbon; PACHO, supercritical fluid extracted HO purified with palm activated carbon; ZHO, supercritical fluid extracted HO purified with zeolite; HO, horse oil.
Notably, the utilization of zeolite led to a significant reduction in hydrocarbons such as dodecane (alkane aroma profile), methane, isocyanato- (cabbage and horseradish-like odor), and pentane, 2,3-dimethyl- (gasoline scent) in ZHO (p<0.05). The microporous nature of the pores in zeolite may demonstrate a high capacity for adsorbing VOCs, making it an effective deodorizing agent. Furthermore, zeolite exhibited an exceptional capability to reduce the levels of sec-butylamine, a contributor to the ammonia and fishy odor observed in CON, to an insignificant amount from its initial level of 16.05 A.U. ×106. Similarly, the level of cyclotetrasiloxane, octamethyl- (without aroma description) was reduced from 108.93 A.U. ×106 to 37.86 A.U. ×106 (p<0.05). Furthermore, the presence of pentane, 2,3-dimethyl (known for its gasoline-like odor) was dramatically reduced from 177.17 A.U. ×106 to 15.91 A.U. ×106 in the ZHO compared to the CON. Interestingly, this study also revealed that zeolite was highly effective at significantly reducing the levels of fruit-like aromatic VOCs in HO, such as nonanal (citrus, lime, or orange-peel scent), octanal (lemon, citrus, or orange-peel scent), and d-limonene (mint, lemon citrus scent; p<0.05). This result is consistent with a recent study by Fuss et al. (2021), demonstrating the role of zeolite as a molecular sieve material, proficient in capturing and eliminating odors in the oil refining process.
Supercritical fluid extraction is a widely adopted method for extracting volatile compounds from various beverage samples and is valuable for volatile compound analysis. This method is particularly suitable for extracting non-polar analytes (Vafaei et al., 2022). Additionally, the inclusion of a small quantity of a polar cosolvent during the process expands its capabilities to include the extraction of more polar analytes from oil (Herrero et al., 2010). Supercritical fluid extraction was found to enhance both the diffusion coefficient and pore penetration properties of extracted oil, which is advantageous for the purification process. The increased pore penetration of HO extracted by supercritical fluid extraction, facilitates the adsorption of impurities by microporous deodorants through van der Waals forces (Castillejos et al., 2012). The deodorization process for oil purification is designed to eliminate VOCs and free fatty acids, ultimately yielding odorless and tasteless oils. AC is commonly utilized to remove impurities such as odors, colors, and tastes from liquids via adsorption (Castillejos et al., 2012; Gibon et al., 2007).
Zeolite is renowned for its exceptional adsorption capacity, particularly for polar molecules such as ammonia and aromatic hydrocarbons, surpassing its performance for non-polar molecules, as supported by recent research (Fuss et al., 2021). Its distinctive molecular porosity, ranging from 0.1 to 1 nm, significantly influences its effectiveness as a deodorant in removing VOCs from oils. The adsorption process is especially efficient for smaller molecules, which are quickly absorbed by zeolite compared with larger molecules. The introduction of zeolite as a deodorant in oil purification allows the effective absorption of undesirable components on the oil surface by employing a combination of physical adsorption and chemisorption mechanisms. This is because of the molecular porosity of zeolite, which provides a larger surface area, leading to an increased adsorption capacity (Fuss et al., 2021). In addition to its deodorizing properties, zeolite possesses catalytic properties that make it valuable in oil purification processes. The tetrahedral units in zeolite create three distinct pore systems, resulting in enhanced catalytic activity compared to that of conventional catalysts. The zeolite surface exhibited a unique combination of properties from both ordinary crystal surfaces and crystal lattices, allowing it to interact strongly with the detected VOCs in the HO. The capability of zeolite to remove carbon from adsorbed molecules continuously increases the hydrogen content in the oil, ultimately leading to improved catalytic efficiency (Morris and Allan, 2017).
PLS-DA is a powerful multivariate statistical tool for analyzing and classifying data with multiple inter-correlated dependent variables (Ruiz-Perez et al., 2020). In this study, the PLS-DA model effectively separated volatile compounds using two principal components (PC1: 32.4%, PC2: 15.1%; Fig. 1). Although the VOCs of CON, ACHO, and PACHO were similar, the VOCs of ZHO were significantly different from those of the other treatments. To further evaluate the contribution of individual VOCs to the PLS-DA classification based on deodorant treatment, variable importance in projection (VIP) scores were calculated (Fig. 2). The VIP score is a relevant index for determining the importance of experimental variables in defining the classification model. In this study, variables with VIP scores above average (>1.2) were considered better indicators for differentiating the effects of the deodorant compounds (Alcantara et al., 2021).
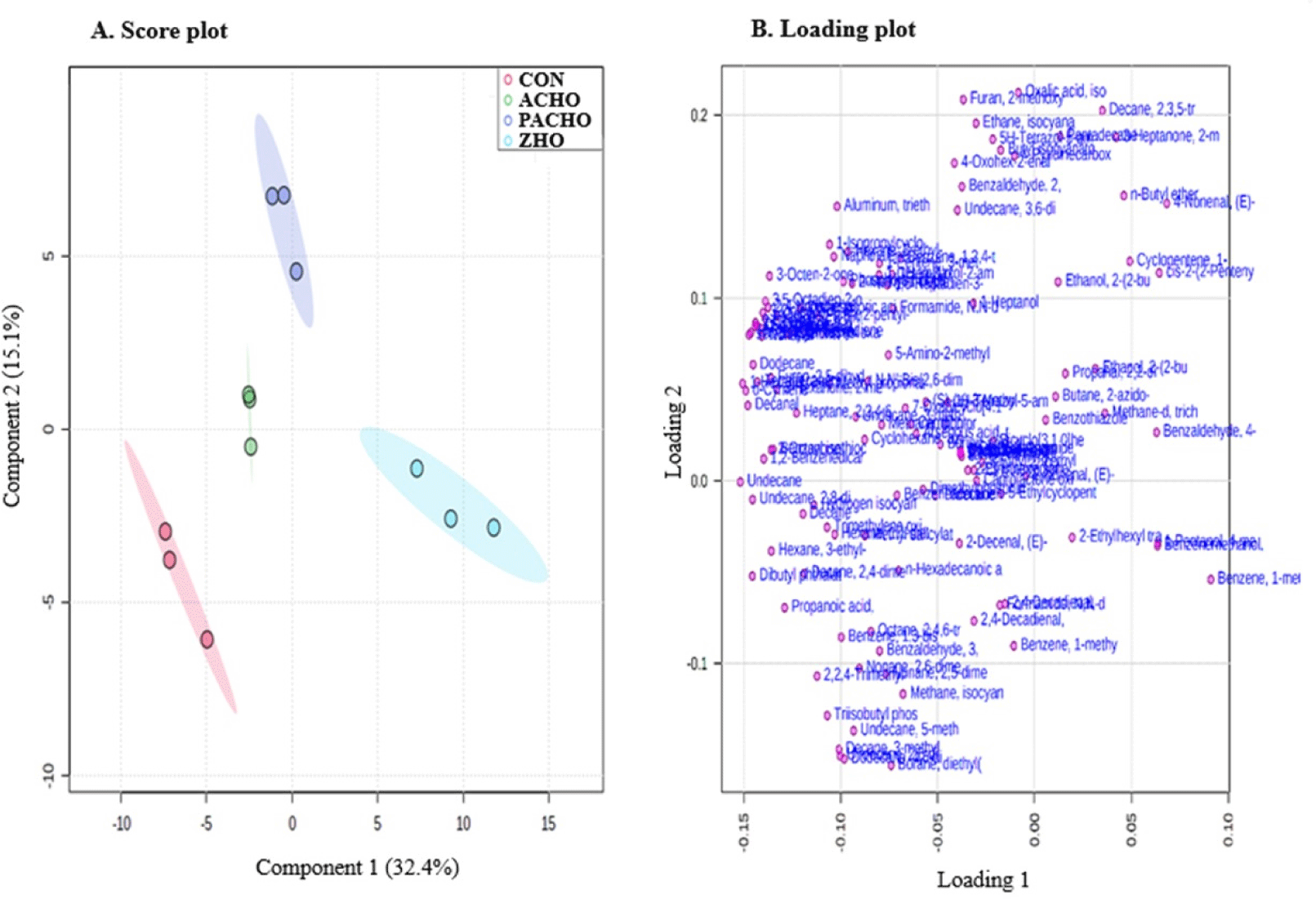
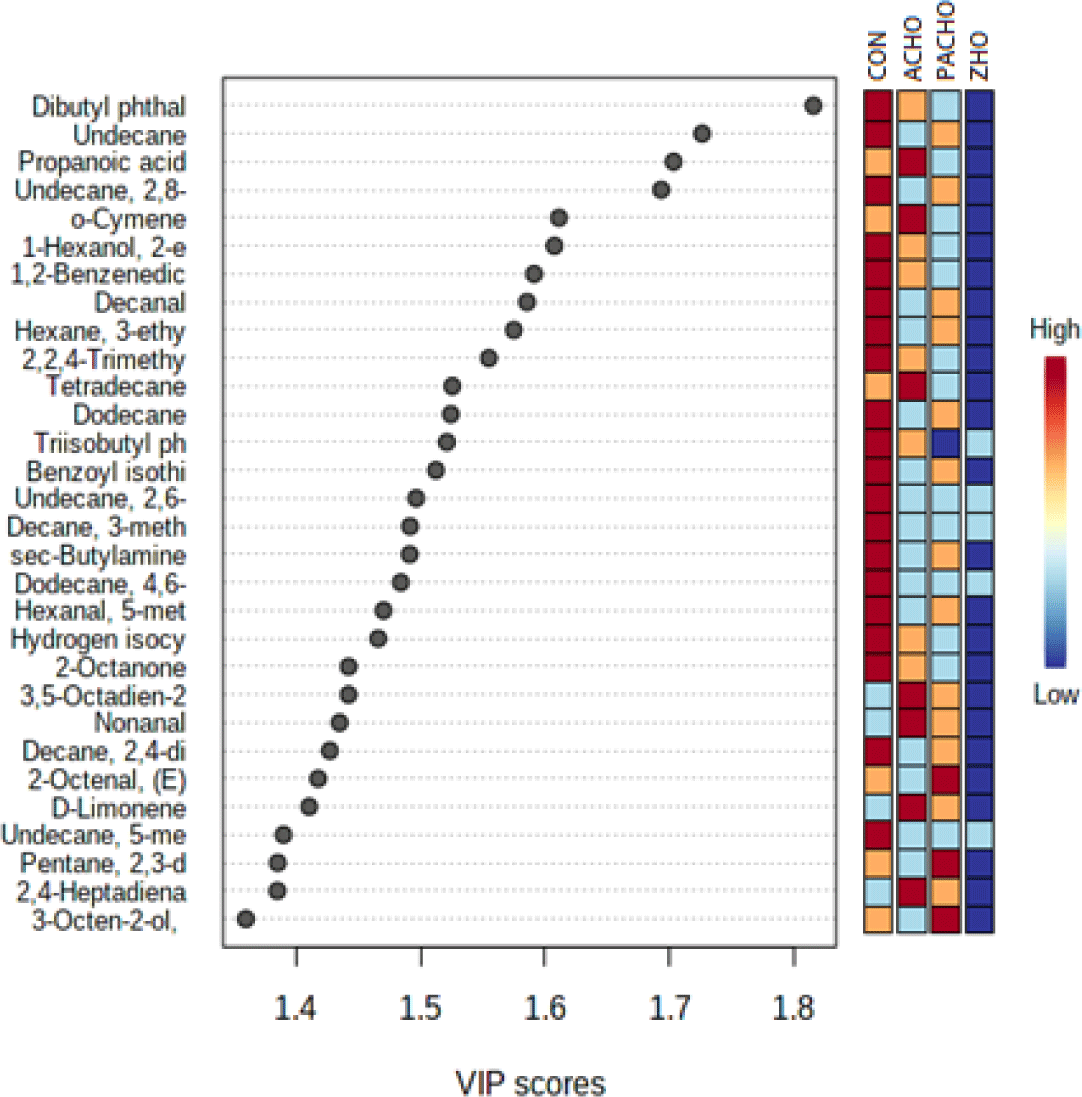
In this study, 30 VOCs were identified as important for discriminating the effects of different deodorants on HO purification (VIP scores>1.2). Dibutyl phthalate, which contributes to a faint odor, had the highest VIP score (>1.8) among the other VOCs and was the most significant contributor to flavor/odor profile discrimination in CON and HO with deodorants. The concentration of dibutyl phthalate was the highest in the HO purified with deodorants. Undecane had the second-highest VIP score and played a role in differentiating the alkane odor of HO, along with other compounds, such as 2,8-dimethyl-, tetradecane, and dodecane (VIP score>1.2). Compounds such as 1-Hexanol, 2-ethyl, nonanal, and D-limonene, with VIP scores higher than 1.2, may contribute to the discrimination of VOCs related to citrus odors in CON and HO with deodorants. The relative contents of VOCs between CON and HO with deodorants were also analyzed and clustered separately in a clustered heatmap (Fig. 3), where the VOCs in ZHO were clustered differently from the other HO with deodorant treatments (ACHO and ZHO). In contrast, the VOCs from CON and PAC-HO were more closely related to each other than those from ACHO and ZHO.
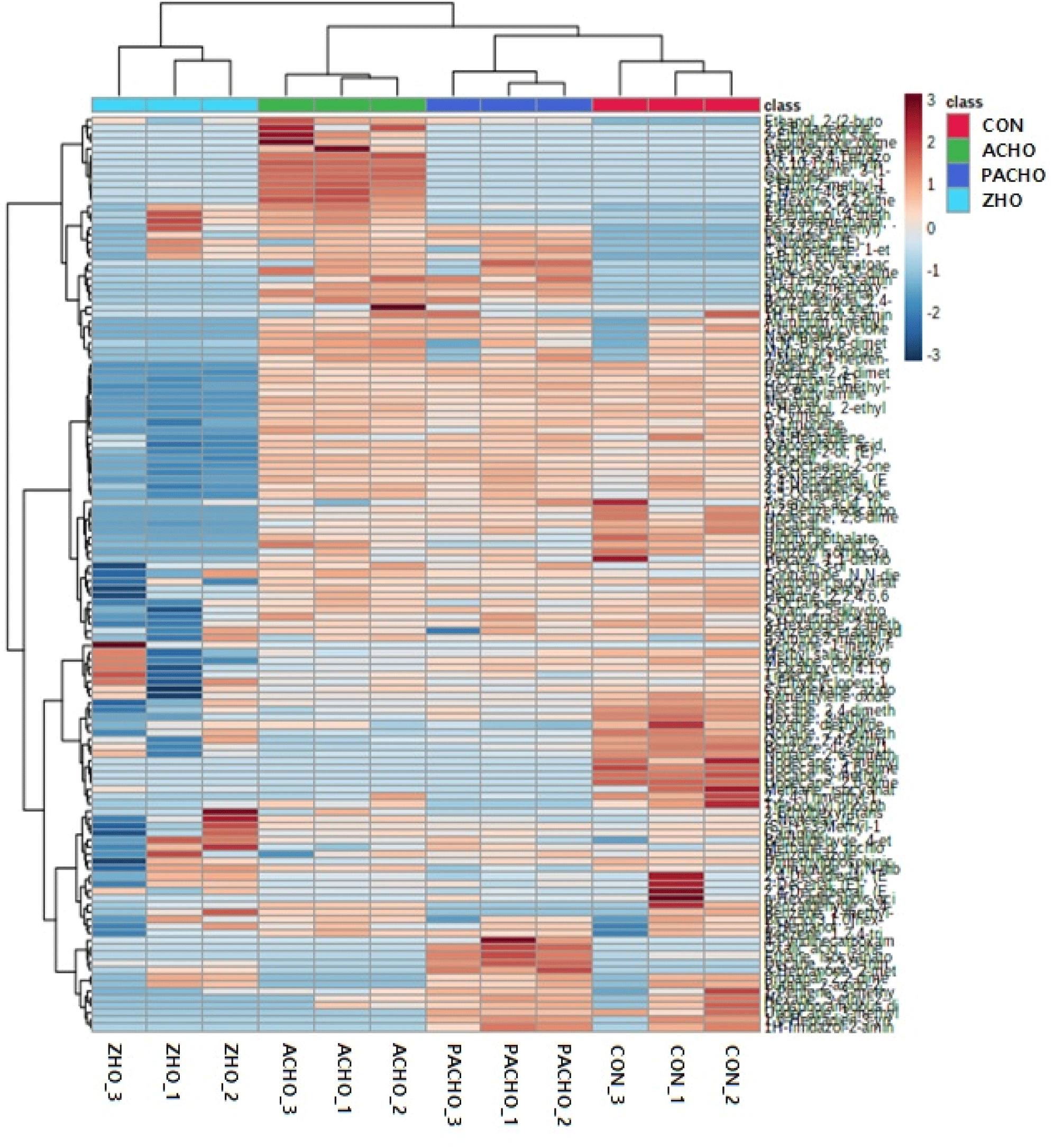
Conclusion
The use of supercritical fluid extraction and various deodorants, including AC, PAC, and zeolite, in the purification of HO did not negatively affect the oil yield, TBARS values, or fatty acid composition. Thirty relevant VOCs, including dibutyl phthalate, undecane, 2,8-dimethyl, tetradecane, dodecane, 1-hexanol, 2-ethyl, nonanal, and D-limonene, showed scores greater than 1.2, indicating their importance in discriminating deodorant effects on HO. Additionally, the use of zeolite as a deodorant resulted in a significant reduction in sec-butylamine and cyclotetrasiloxane, octamethyl-, as well as the total counts of VOCs in purified HO (p<0.05), which may be beneficial for further use. This study provides preliminary data on the characteristics of HO purified using these deodorants; however, further research is needed to understand the mechanisms underlying their effects on VOCs and the changes in off-odor-related VOCs produced before, during, and after the purification process.