Introduction
In the late 1970s, Danish researchers Hans Olaf Bang and Jørn Dyerberg discovered that the Inuit had a very low incidence of cardiovascular disease (CVD) despite their diet of seal and whale blubber and oily fish (Dyerberg and Bang, 1979). Additionally, the Inuit were found to have nearly 2-fold longer bleeding times than Danes. The food consumed by the Inuit contains a high content of ω-3 polyunsaturated fatty acids (PUFAs), which replace ω-6 PUFAs and reduce platelet aggregation.
In 1980, an epidemiological survey on the anti-arteriosclerotic effect of fish oil was conducted at Chiba University in Japan (Hirai, 1985). Fishermen with high fish intake were compared with rural residents with low fish intake, and the fishermen’s eicosapentaenoic acid (EPA) mean intake was 2,600 mg/day, approximately three times higher than rural residents’ EPA mean intake. Fishermen had lower mortality rates from ischemic heart disease and CVD compared to rural residents. They also had lower platelet aggregation and blood viscosity, and triglyceride levels were about 40% lower.
The rural area population of the Nenets Autonomous Okrug (NAO) in Arkhangelsk city, Russia, eats lean reindeer meat and local cold-water white fish rich in ω-3 PUFAs. Therefore, their serum lipid profiles were investigated and compared with those of the urban area population (Petrenya et al., 2012). Compared to the urban population, the NAO rural population has decreased total cholesterol, low-density lipoprotein cholesterol, very low-density lipoprotein cholesterol, and triglyceride. In contrast, high-density lipoprotein cholesterol has increased, showing positive health results. In Siberia and Chukotka, Chukchi residents who consumed a lot of sea fish and fish products had higher blood EPA and docosahexaenoic acid (DHA) levels than residents of the urban area of Novosibirsk city (Rezvukhin et al., 1996).
Fatty acid intake varies depending on human lifestyle (Fig. 1; Simopoulos, 2016). As humans began to engage in agriculture in earnest, domestication of animals occurred, and many changes occurred in the fatty acid intake [linoleic acid (LA) and alpha-linolenic acid (ALA)] of humans and animals. During the Paleolithic and Neolithic periods, when food was mainly obtained through hunting, ω-6 PUFAs were consumed at a lower rate than in modern times. Livestock that consumed grains had increased LA content in meat (Naughton et al., 2016). Since the Industrial Revolution Era, the intake rate of ω-6 PUFAs has gradually accelerated. In the Late Paleolithic period, 8.84 (g/day) of LA and 12.6 (g/day) of ALA were consumed, and the ω-6/ω-3 ratio is estimated to be 0.79 (Simopoulos, 2016). Since 1960, consumption of vegetable oils such as rapeseed (canola), soybean, sunflower, and corn oils has increased 1,000-fold in the US, contributing to an increase in obesity, type 2 diabetes, nonalcoholic steatohepatitis, and other inflammatory disorders. Currently, ω-6 PUFA-rich vegetable oils, such as soybean and canola, are widely used as cooking oils in homes and restaurants worldwide (Yamashima et al., 2020). In the Western diet, as the intake of high-fat diet (HFD) increases, the intake of ω-3 PUFAs decreases, and the intake of ω-6 PUFAs increases, resulting in a ω-6/ω-3 ratio of 10:1 to 25:1 (Weiser et al., 2016).
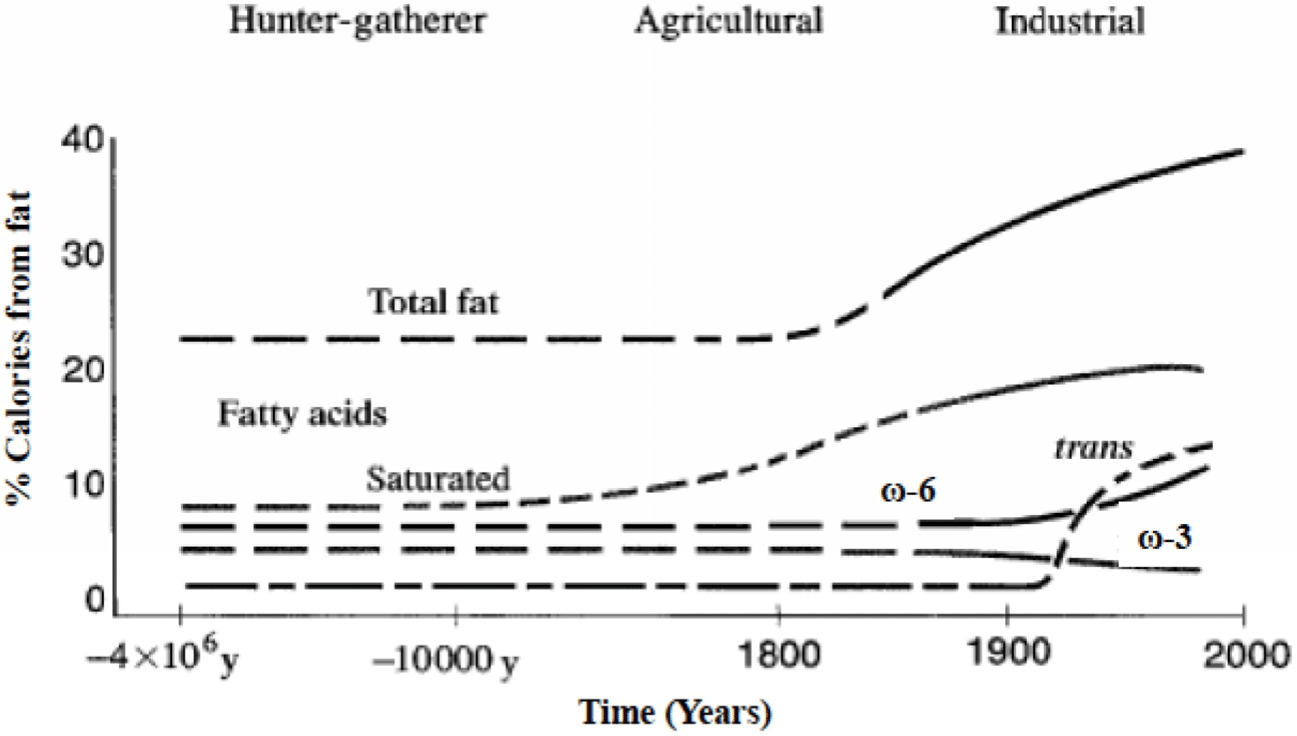
High-ratio ω-6/ ω-3 PUFAs are essential in increasing obesity through the hyperactivity of AA eicosanoid metabolites and cannabinoid system. Balancing the LA/ALA ratio is integral for obesity prevention and human health. Obesity is defined by the World Health Organization (WHO) as abnormal or excessive fat accumulation that can pose a risk to health (WHO, 2022). In 2022, 1 in 8 people in the world will be obese, and 2.5 billion adults (aged 18 and over) will be overweight (43%), of whom 890 million will be obese (16%). The passive accumulation of excess weight does not cause obesity but is a disorder of the energy homeostasis system. Red blood cells from obese patients have high ω-6 PUFAs and low ω-3 PUFAs (Sot et al., 2022). CVDs affect one in four people in Europe and one in three in the United States (Dyńka et al., 2023). CVD is the most common global cause of death, with an average of 17.9 million people dying from this disease worldwide each year. In 2020, the number of deaths due to CVD exceeded 19 million, an increase of 18.7% compared to 2010, and approximately 23.3 million people are expected to die due to CVD by 2030. Diet is among the most critical risk factors in CVD prevention and treatment. Fish oil, rich in ω-3 PUFAs, has immunomodulatory effects and can inhibit the production of proinflammatory cytokines. ω-3 PUFAs (EPA and DHA) have anti-obesity and anti-inflammatory effects, and the European Food Safety Authority’s average intake recommendation for human health is 250 to 500 mg/d (Avallone et al., 2019). However, most Western diets lack the recommended intake of ω-3 PUFAs, so ω-3 PUFAs supplementation is essential. This can be achieved by directly consuming fish, fish oils, krill oils, flaxseed oil, and leafy vegetables rich in ω-3 PUFAs or meat, milk, and eggs fortified with ω-3 PUFAs.
In the European Union (EU), foods containing 300 mg of ALA or 40 mg of combined EPA and DHA per 100 g and 100 kcal can be labeled as “Source of ω-3 fatty acids”, and foods containing 600 mg or 80 mg can be labeled as “High in ω-3 fatty acids” (Commission Regulation EU, 116/2010). The primary source of ω-3 PUFAs is fish oil, and the wholesale price averages 14 dollars per kg (Benvenga et al., 2022). The global market for ω-3 PUFAs is estimated to be worth 2.10 billion United States dollars (USD) in 2020, and with an annual growth rate of 7.8%, it is expected to reach 3.61 billion USD in 2028.
Sources of ω-3 and ω-6 Polyunsaturated Fatty Acids
LA and ALA are essential fatty acids in animals and must be consumed through diet or supplementation (Bermudez Menendez de la Granda and Sinclair, 2009). Fish accumulate ω-3 fatty acids derived from microalgae (phytoplankton) and are particularly rich in EPA and DHA. ALA is a ω-3 fatty acid abundant in microalgae, fish, krill oils, flaxseed oils, green leafy vegetables, and animal fat, especially in grass-fed animals. LA is a ω-6 fatty acid abundant in grains, meats, and the seeds of most plants (Table 1; Amjad Khan et al., 2017).
Dietary sources | ALA (18:3, ω-3) | EPA (20:5, ω-3) | DHA (22:6, ω-3) | LA (18:2, ω-6) | AA (20:4, ω-6) | ω-6/ω-3 ratio | Units | References | |
---|---|---|---|---|---|---|---|---|---|
Microalgae | Schizochytrium sp. | 0.97 | 1.90 | 202.62 | 0.15 | 0.59 | 0.0:1 | mg/g DP | Xu et al. (2021) |
Fishes | Salmon (raw) | - | 1.01 | 0.94 | - | - | 0.0:1 | g/100 g | USDA (2015) |
Herring (raw) | - | 0.97 | 1.18 | - | - | 0.0:1 | g/100 g | USDA (2015) | |
Plant oils | Flaxseed | 53.4 | - | - | 14.3 | - | 0.3:1 | g/100 g | USDA (2015) |
Perilla | 62.6 | - | - | 15.4 | - | 0.3:1 | g/100 g | Kawamura et al. (2022) | |
Soybean | 6.79 | - | - | 50.42 | - | 7.4:1 | g/100 g | USDA (2015) | |
Corn | 1.16 | - | - | 53.23 | - | 45.9:1 | g/100 g | USDA (2015) | |
Grains | Wheat | 4.04 | - | - | 59.09 | - | 14.6:1 | g/kg total FA | Ozturk et al. (2012) |
Barley | 4.52 | - | - | 56.65 | - | 12.5:1 | g/kg total FA | Ortiz et al. (2021) | |
Pastures | Grass hey | 61.62 | - | - | 14.09 | - | 0.2:1 | g/kg total FA | Krusinski et al. (2023) |
Grass silage | 54.27 | - | - | 12.24 | - | 0.2:1 | g/kg total FA | Mierlita et al. (2022) | |
Alfalfa hay | 17.95 | - | - | 8.25 | - | 0.5:1 | g/kg total FA | Mierlita et al. (2022) | |
Animals products | Beef (lean, sirloin) | 4 | 0 | 0 | 33 | 5 | 9.5:1 | g/kg total FA | USDA (2011) |
Pork (lean, trimmed) | 4 | 0 | 0 | 95 | 10 | 26.3:1 | g/kg total FA | USDA (2011) | |
Chicken (light meat) | 7 | 3 | 10 | 179 | 26 | 9.3:1 | g/kg total FA | USDA (2011) | |
Milk (whole) | 15 | 0 | 0 | 23 | 0 | 1.5:1 | g/kg total FA | USDA (2011) | |
Egg (yolks) | 4 | 0 | 4 | 134 | 17 | 18.9:1 | g/kg total FA | USDA (2011) |
Microalgae are the earliest producers of very long-chain PUFAs in the marine food chain (Amjad Khan et al., 2017). ω-3 PUFAs synthesized in microalgae are biomagnified in the food web, reaching higher trophic levels. Therefore, ω-3 PUFAs synthesized in microalgae accumulate in the tissues of marine fish such as salmon and herring. On the other hand, animals that consume cereal-based feed accumulate ω-6 PUFAs in more significant proportions. However, animals that consume flaxseed oils and green leafy vegetables can also produce beef, pork, and chicken, accumulating ω-3 PUFAs. ω-3 PUFAs derived from external sources are incorporated into lipoproteins and membrane phospholipids in the body. DHA is highly accumulated in neuronal membranes (brain), retina photoreceptors (eyes), and muscles and is involved in the development and function of these organs (Fig. 2; Bae et al., 2023).
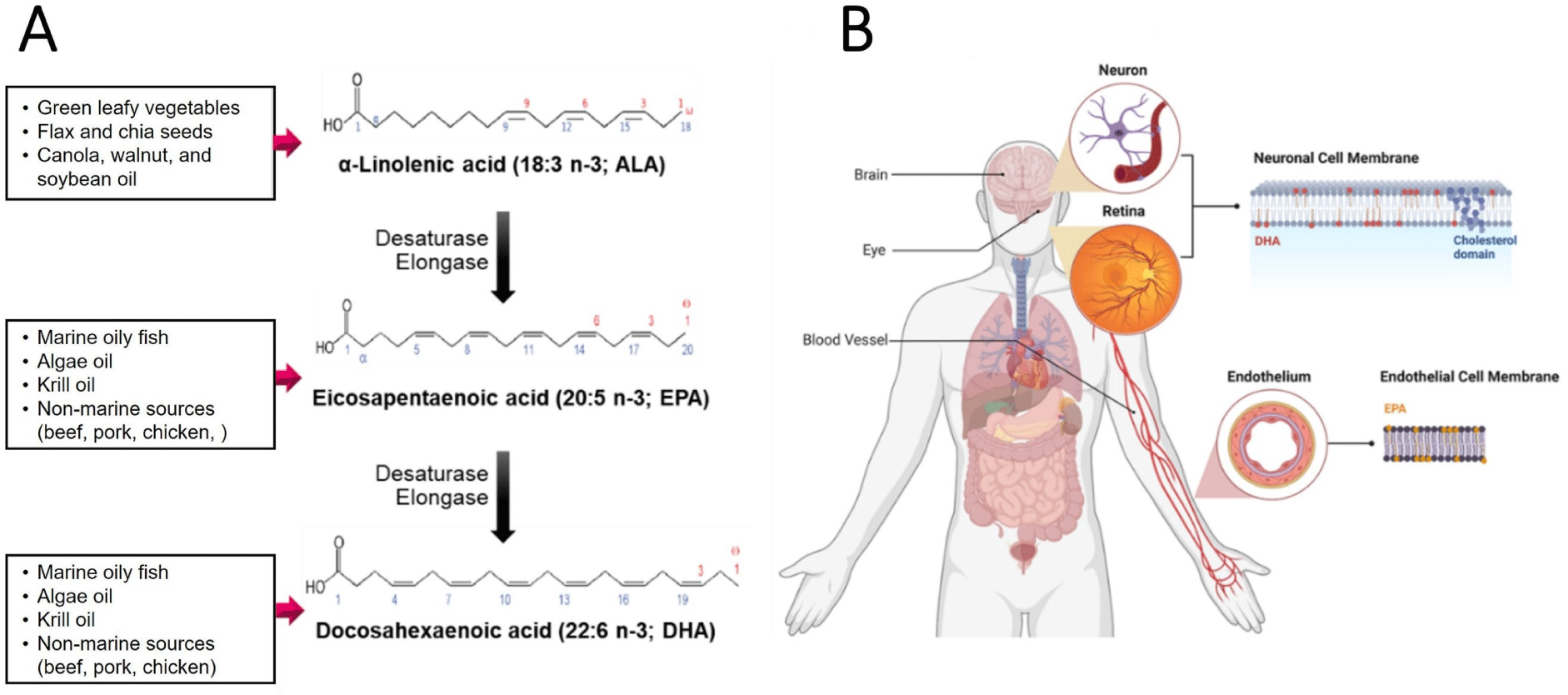
Metabolism of ω-3 and ω-6 Polyunsaturated Fatty Acids
The synthesis of fatty acids begins with acetyl-CoA in the cytoplasm, and two carbons are added to the fatty acid chain through malonyl-CoA (Fig. 3; Qin et al., 2023). After eight cycles, the synthesized stearic acid (SA, C18:0) is transferred to the endoplasmic reticulum (ER) and begins the first unsaturation step. SA is desaturated by Δ9 desaturase to produce oleic acid (OA, C18:1Δ9), and OA is desaturated by Δ12 desaturase to produce ω-6 LA (C18:2Δ9,12). Afterward, LA produces ω-3 ALA (C18:3Δ9,12,15) by Δ15 desaturase.
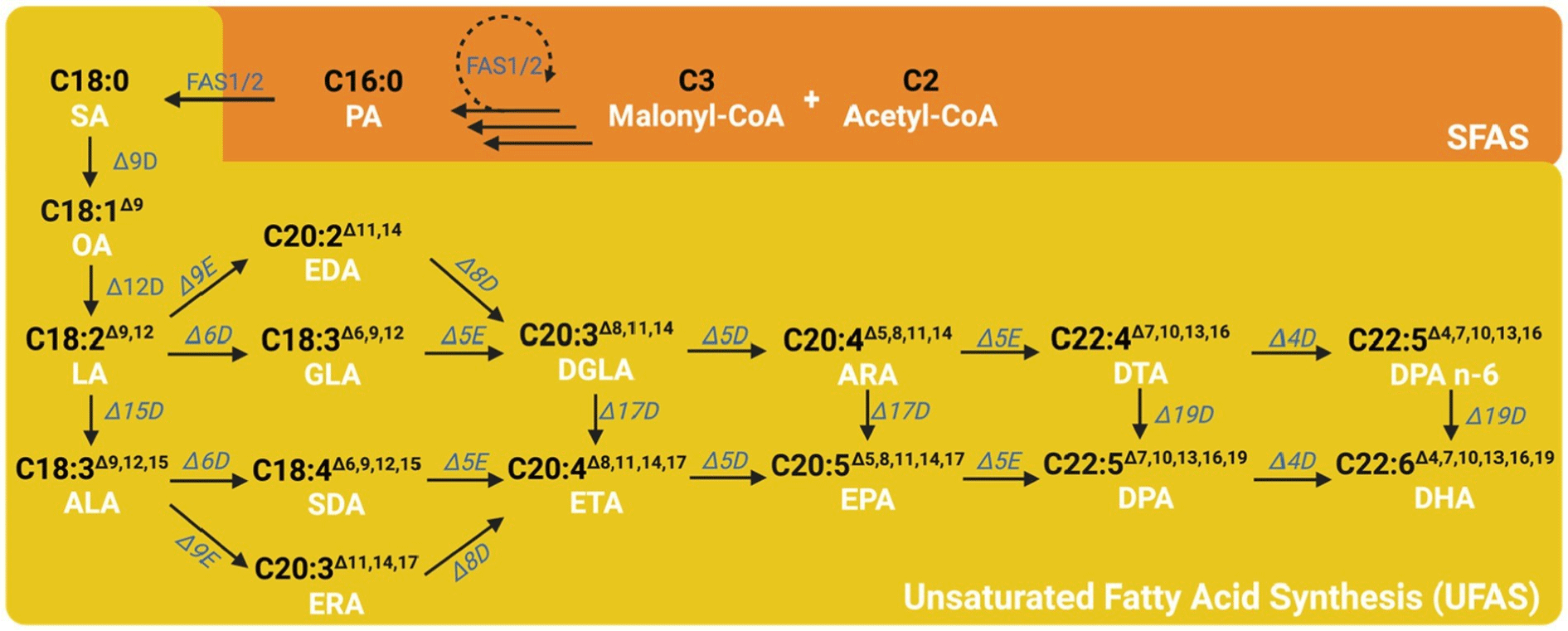
Almost all higher plants, algae, and some fungi have Δ12-desaturases that convert OA to LA and Δ15-desaturases that convert LA to ALA (Ruiz-Lopez et al., 2015). However, animals and humans do not possess these desaturases, so LA and ALA are the essential FAs. The difference in the double bond between LA and ALA causes them to play different roles in inflammation and metabolism (D’Angelo et al., 2020). LA and ALA are synthesized into ARA (AA) and EPA by Δ6 desaturase, Δ5 desaturase, Δ4 desaturase, and elongases in the ER of cells, and then into DHA in the peroxisome (Salem et al., 2015). LA and ALA are utilized competitively by these enzymes. When the intake of LA is high, the conversion rate of EPA and DHA from ALA decreases; when the intake of ALA is high, the conversion rate of ARA (AA) from LA decreases (Ailhaud et al., 2006). However, although humans and animals can synthesize both EPA and DHA, their conversion rates do not meet nutritional demands, so they must be supplied from external sources such as microalgae, fish, and flaxseed oils.
The key enzymes metabolizing LA and ALA are Δ6 desaturases and Δ5 desaturases, encoded by the fatty acid desaturases (FADS) 1/2 genes. Δ5 desaturases are encoded by the FADS1 gene and convert LA and ALA into long-chain PUFAs (such as ARA and EPA). FADS1 rs174550 genotypes (TT and CC) strongly regulate plasma lipid PUFA composition. The FADS1 haplotype has 43% and 24% lower plasma proportions of AA and DHA, respectively, compared to the homozygous haplotype. According to estimates from the Human Genome Diversity Project-Centre denture du Polymorphism Humain (HGDP-CEPH) panel, haplotype A has a frequency of 1% in African populations, 97% in native Americans, and 25%–50% in Europeans and Asians (Ameur et al., 2012). People with the FADS1 rs174550-TT genotype have increased LA phospholipids and inflammatory adipose tissue gene expression when consuming an LA-enriched diet compared to people with the FADS1 rs74550-CC genotype. Humans of the FADS1 rs74550-CC genotype play a protective role against LA-induced inflammation through the regulation of AA-derived eicosanoids biosynthesis (Vaittinen et al., 2022). Therefore, an approach that considers the variation of FADS should be applied to personalized dietary counseling.
PUFAs, LA, and ALA exhibit pro-inflammation and anti-inflammation effects through metabolic and hydrolyzing processes in the body (Fig. 4; D’Angelo et al., 2020; Ishihara et al., 2019). LA and ALA are converted into AA, EPA, and DHA, which are then converted into bioactive lipid mediators by cyclooxygenases (COXs), lipoxygenases (LOXs), and cytochrome P450 monooxygenases to perform biological actions. ω-6 AA is released from membrane phospholipids by inflammatory stimuli and phospholipase A2 (PLA2). The released AA is converted into prostaglandins (PGs), leukotrienes (LTs), and lipoxins by COXs and LOXs, showing pro-inflammation effects. On the other hand, ω-3 EPA and DHA are converted into metabolites (3-series PGs, 5-series LTs) and anti-inflammatory mediators (resolvins, protectins, maresins, etc.) at inflammation sites and exhibit anti-inflammatory effects. Additionally, ω-3 PUFAs (EPA, DHA) exhibit anti-inflammatory effects through substrate and receptor competition with ω-6 AA.
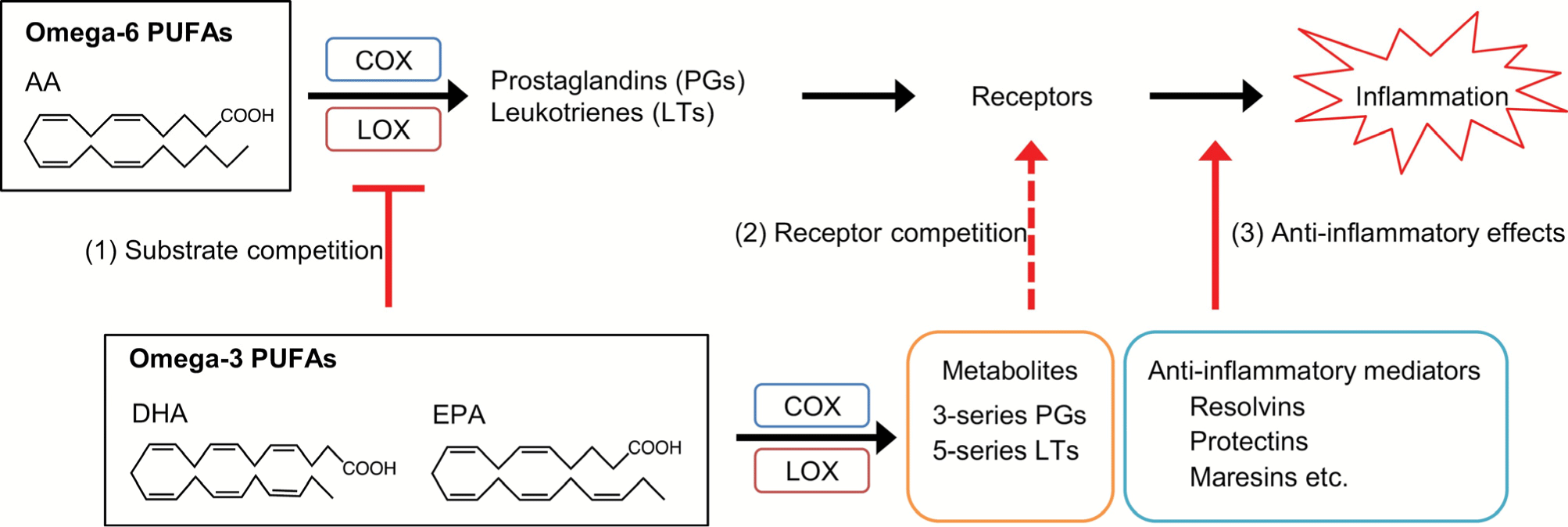
Corticosteroids exert anti-inflammatory effects by inhibiting the activity of PLA2, which releases ω-6 AA from cell membranes (Fig. 5; Kousparou et al., 2023; Rice et al., 2017). Zileuton acts as a 5-LOX inhibitor, and Montelukast acts as a LT receptor antagonist, showing anti-inflammatory effects. Nonsteroidal anti-inflammatory drugs, such as aspirin (acetylsalicylate), are COX irreversible inhibitors and exert anti-inflammatory effects by inhibiting the production of prostanoids (Bindu et al., 2020).
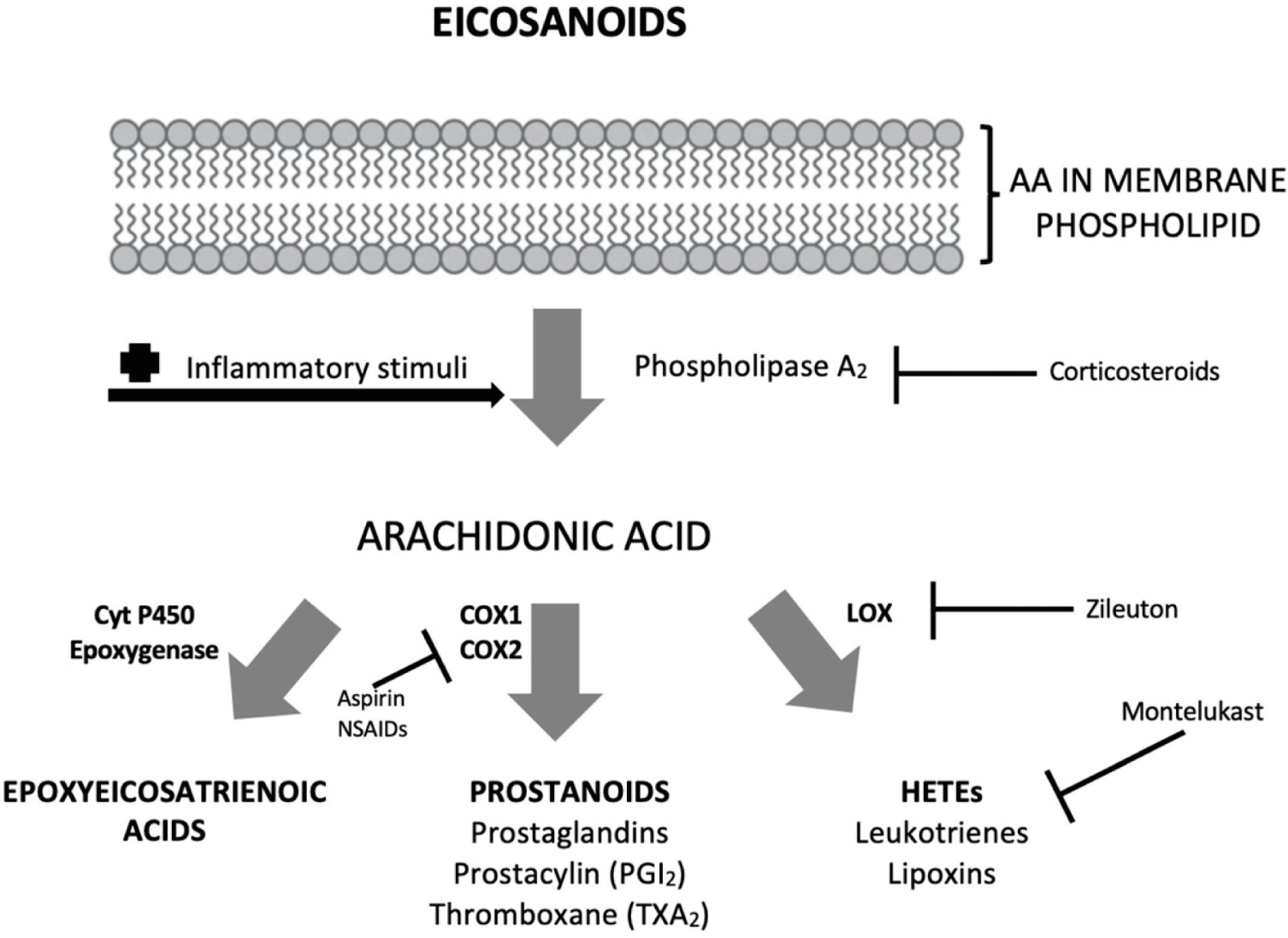
Excessive cellular AA (from dietary LA intake) can stimulate adipocyte differentiation through several interconnected pathways (Fig. 6; Naughton et al., 2016). AA (20:4 ω-6) can be converted to prostacyclin via prostaglandin H2 (PGH2), but this reaction is reduced in the presence of cellular ALA. Prostacyclin stimulates the peroxisome proliferator-activated receptor (PPAR) family, leading to PPARγ activation and adipocyte differentiation. Prostacyclin can stimulate adipocyte differentiation through the CCAAT-enhancer binding protein family (CEBPβ and CEBPδ). Additionally, AA can activate PPARγ by increasing cyclic adenosine monophosphate (cAMP) production and protein kinase A activity (Petersen et al., 2003). The cAMP process is inhibited by a COX inhibitor (aspirin). ALA’s metabolites, EPA (2O:5 ω-3) and DHA (22:6 ω-3), inhibit the production of PGH2 and cAMP by AA (Massiera et al., 2003). Another mechanism by which LA causes obesity is the endocannabinoid (EC) pathway. The primary mediators of ECs include N-arachidonoylethanolamine (anandamide) and 2-arachidonyl glycerol derived from LA, which increase appetite and food intake by activating cannabinoid receptors (CB1 and 2). Conversion of the ω-6 and ω-3 PUFAs share the same biochemical pathway involving desaturation and elongation reaction processes. LA and ALA compete for metabolic enzymes. Therefore, balancing LA/ALA ratios is very important for obesity prevention and human health (Wang and Wang, 2023).
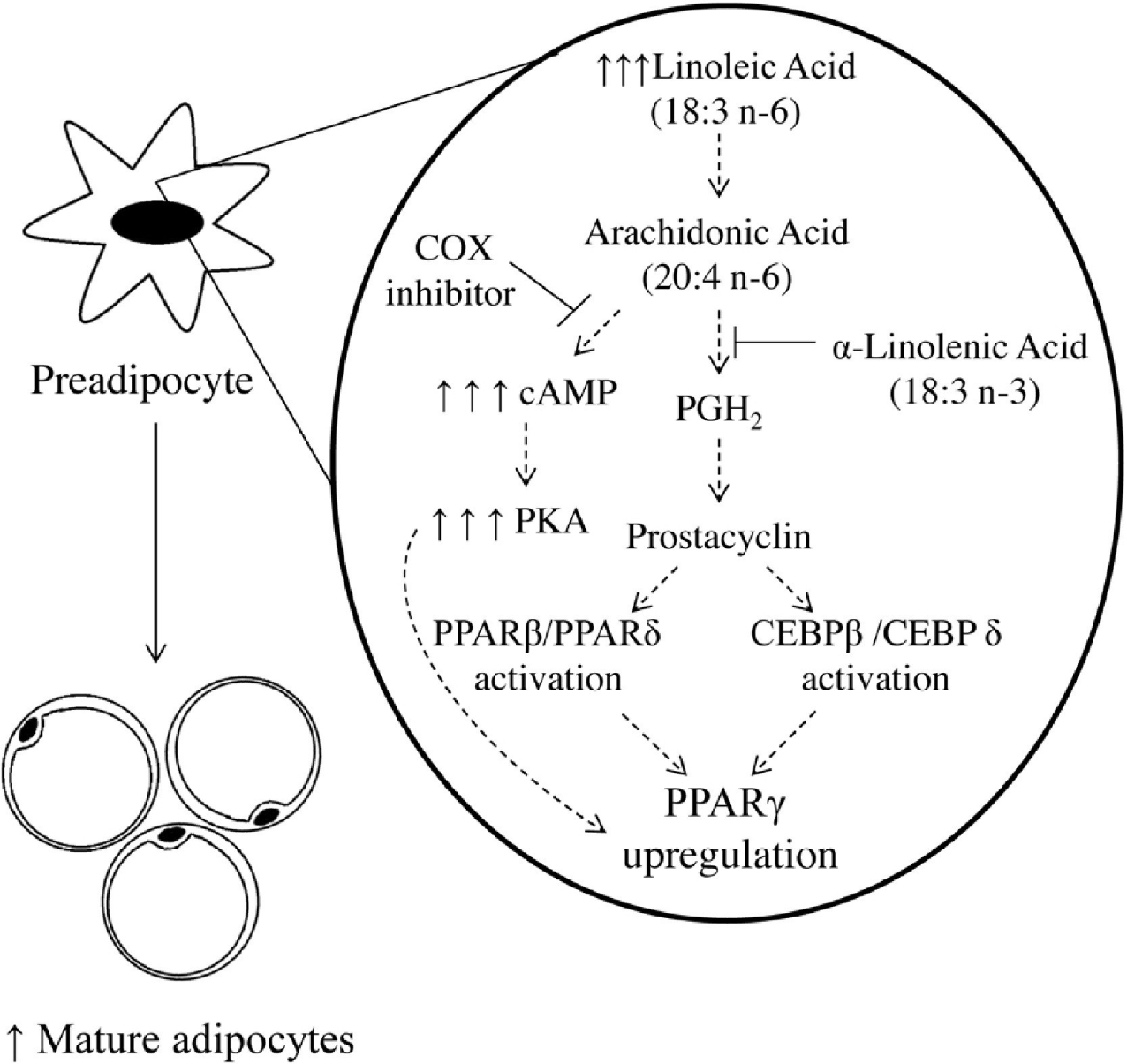
Current Dietary Guidelines for Americans (DGA) recommend replacing saturated fat acids (SFAs) intake with PUFAs and monosaturated fatty acids (MUFAs). Additionally, modern Western diets are already rich in ω-6 PUFAs, which increases the risk of chronic disease. Therefore, to resolve this problem, increasing intake of ω-3 PUFAs rather than ω-6 PUFAs is beneficial for obesity and related liver diseases caused by HFDs. For instance, in a study on the interventional effect of different dietary PUFAs on obesity, C57BL/6J mice were fed a HFD for ten weeks to induce obesity. Then, the obese mice were divided into three groups and fed one of the following three diets for an additional ten weeks (Fig. 7; Hao et al., 2023). The three groups consisted of the HF group, the HF+ω-6 group (substituted half of SFA with ω-6 PUFAs), and the HF+ω-3 group (substituted half of SFA with ω-3 PUFAs). In the HF+ω-6 group, body weight and fat mass continued to increase like the HF group, but in the HF+ω-3 group, body weight and fat mass decreased significantly, and the proportion of relative lean mass/body weight increased.
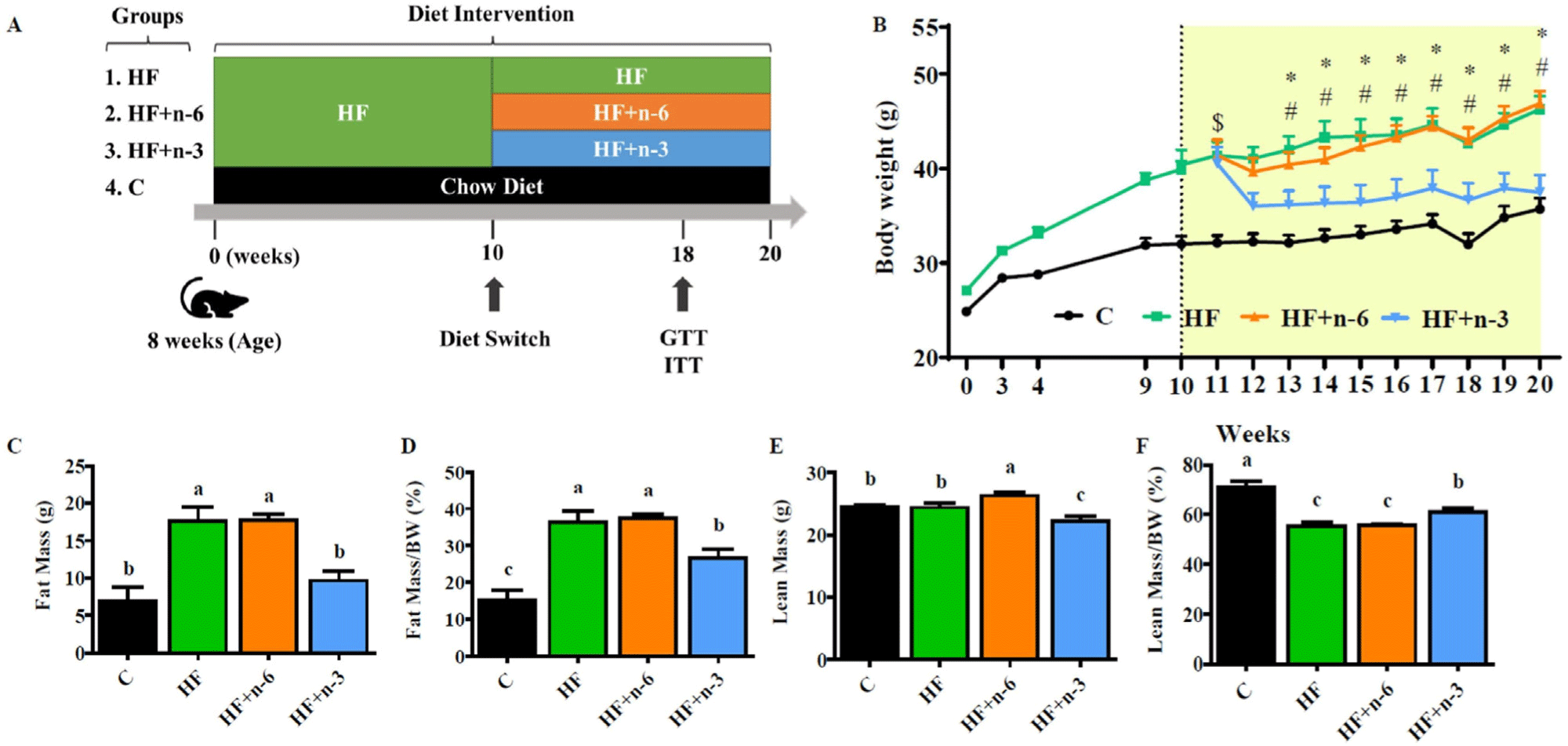
Approaches to Enhance ω-3 Polyunsaturated Fatty Acids in Animal Food
Fatty acids are major components of lipids, forming the lipid bilayer of cells and organelles (De Carvalho and Caramujo, 2018). Animal fatty acids originate from de novo or exogenous sources, and certain fatty acids are synthesized from glucose or through the metabolism of lipid precursors. Ruminant animals have a lower content of ω-6 PUFAs compared to non-ruminant animals (Table 1). Different food sources have different types and contents of fatty acids, which are absorbed into the body and esterified and metabolized to form fatty acids or lipids (Kousparou et al., 2023). Different cells have different fatty acid compositions and different membrane fluidities and permeabilities. Membrane phospholipid fatty acids are mostly hydrocarbon chains of 12 to 24 carbon atoms. ALA sources mainly used in animal feed include linseed oil, rapeseed oil, microalgae, and fish oil (Irawan et al., 2022).
In a mouse model, soybean oil, corn oil, and a mixture of flaxseed and canola oil were fed at LA/ALA ratios of 1:1, 7:1, and 44:1, respectively, for 14 weeks (Fig. 8; Su et al., 2016; Watanabe and Tatsuno, 2020). And the levels of AA, EPA, and DHA in the liver, brain, and muscle were examined. When the LA/ALA ratio was 1:1, there was no difference in AA, EPA, and DHA levels in the liver, brain, and muscle. On the other hand, when the LA/ALA ratios were 7:1 and 44:1, AA levels in the liver and muscle increased, and EPA and DHA levels decreased. However, there was no significant difference in the levels of AA and DHA in the brain when the LA/ALA ratio was 1:1, 7:1, and 44:1. Δ6 desaturase competitively uses LA and ALA to synthesize AA and EPA, and Δ4 desaturase uses EPA to synthesize DHA. In human hepatoma cells, EPA and DHA formation is highest when the LA/ALA ratio is 1:1, with an EPA conversion rate of 17% and a DHA conversion rate of 0.7% (Harnack et al., 2009). The conversion of ALA to EPA and DHA in the body is minimal, with the conversion rate of EPA being 7.0%–21% and that of DHA being 0.01%–1% (Saini et al., 2021). Therefore, increasing ALA intake while reducing LA intake is an effective way to improve ω-3 PUFA status.
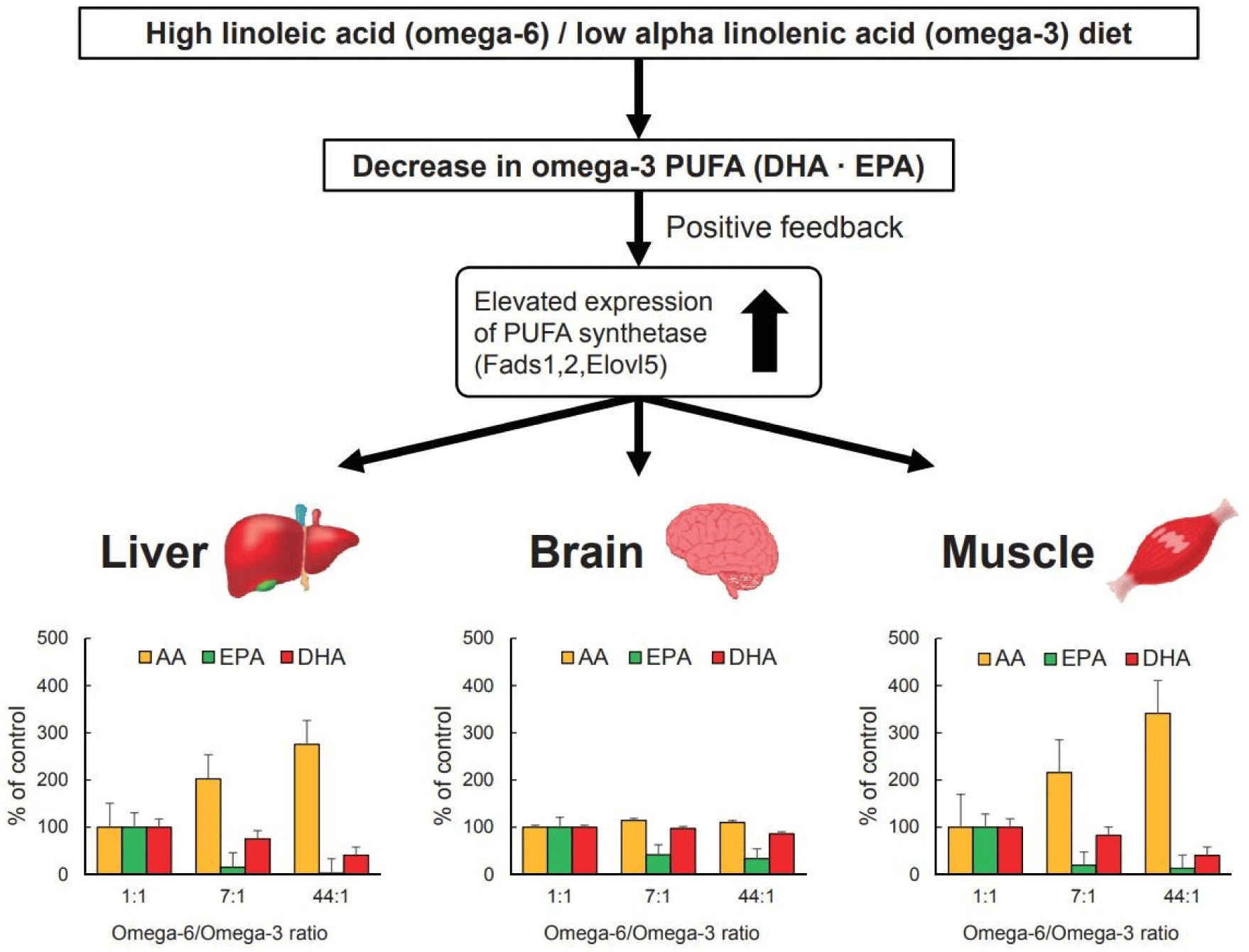
Additionally, among ω-3 PUFAs, DHA supplementation is the most effective way to increase ω-3 DHA levels in the body (Harnack et al., 2009). The human brain is a lipid-rich organ that consumes up to 20% of daily energy intake despite making up only 2% of body weight. More than 50% of the brain’s dry weight (DM) comprises lipids, 10%–20% of brain total lipids are ω-3 PUFAs, and more than 90% of ω-3 PUFAs are DHA (Weiser et al., 2016). DHA is a precursor of bioactive mediators that resolve the brain’s inflammation of neurons, microglia, and endothelial cells. However, the brain’s capacity to synthesize DHA is deficient, so it absorbs DHA from circulating lipid pools to maintain homeostatic levels. DHA transport from plasma to the brain is regulated by the blood-brain barrier (Li et al., 2023). Endogenous synthesis of EPA and DHA from ALA is insufficient to meet the body’s physiological needs and must be supplied from external sources such as microalgae, fish, and fish oils (Huang et al., 2020). In mice, a diet with a low LA/ALA ratio significantly increases the levels of ALA and DHA in erythrocytes, liver, epididymal fat, spleen, brain, heart, and gastrocnemius (Wang and Wang, 2023).
According to the United States Department of Agriculture (USDA, 2011), the PUFA of regular egg yolk has an ω-6/ω-3 ratio of 19.9, while the PUFA of egg yolk from free-range chickens has an ω-6/ω-3 ratio of 1.3 (Simopoulos and Salem, 1992). It is a common strategy to increase ω-3 PUFAs in eggs by dietary supplementation of ALA, EPA, and DHA to laying hens. The total fatty acids (TFAs) of yolk lipids are SFAs at 30%–35% and MUFAs at 40%–45%, which has an appropriate SFA/MUFA ratio, but PUFAs are very low at 20%–25% (Nimalaratne and Wu, 2015). Eggs from laying hens fed standard feed mixtures contained a higher proportion of ω-6 PUFAs and a lower proportion of ω-3 PUFAs. The feeding mixtures of laying hens consisted of 5% soybean oil in the control group and 5% fish oil and linseed oil (1.5%+3.5%) in the treatment group (Fig. 9; Radanović et al., 2023). The egg yolk of the control group (5% soybean oil) contained 1,553.51 mg/100 g of ω-6 PUFAs, 149.88 mg/100 g of ω-3 PUFAs, and 10.36 of ω-6/ω-3 ratio. In the treatment group (5% fish oil and linseed oil), 11,127.71 mg/100 g of ω-6 PUFAs and 598.59 mg/100 g of ω-3 PUFAs accumulated, and 1.8 of ω-6/ω-3 ratio. In this study, compared to the control group, ALA increased by 5.6 times, DHA increased by 2.4 times, and the ω-6/ω-3 ratio decreased by 5.5 times in the treatment group. ω-3 PUFAs enriched eggs had improved atherogenic index, thrombogenic index, and hypo/hypercholesterolemic indexes of egg yolks compared to conventional eggs. They increased ω-3 ALA content and egg health index of examinees’ blood serum. Supplementation of broiler chicken feed with flaxseed oil as a ω-3 source and the antioxidant quercetin, which limits lipoperoxidation, increased the deposition of ω-3 PUFAs within breast muscles and decreased the ω-6/ω-3 ratio (Sierżant et al., 2022). These results provide a strategy to suppress the occurrence of unpleasant taste and smell while increasing the content of ω-3 PUFAs in poultry meat.
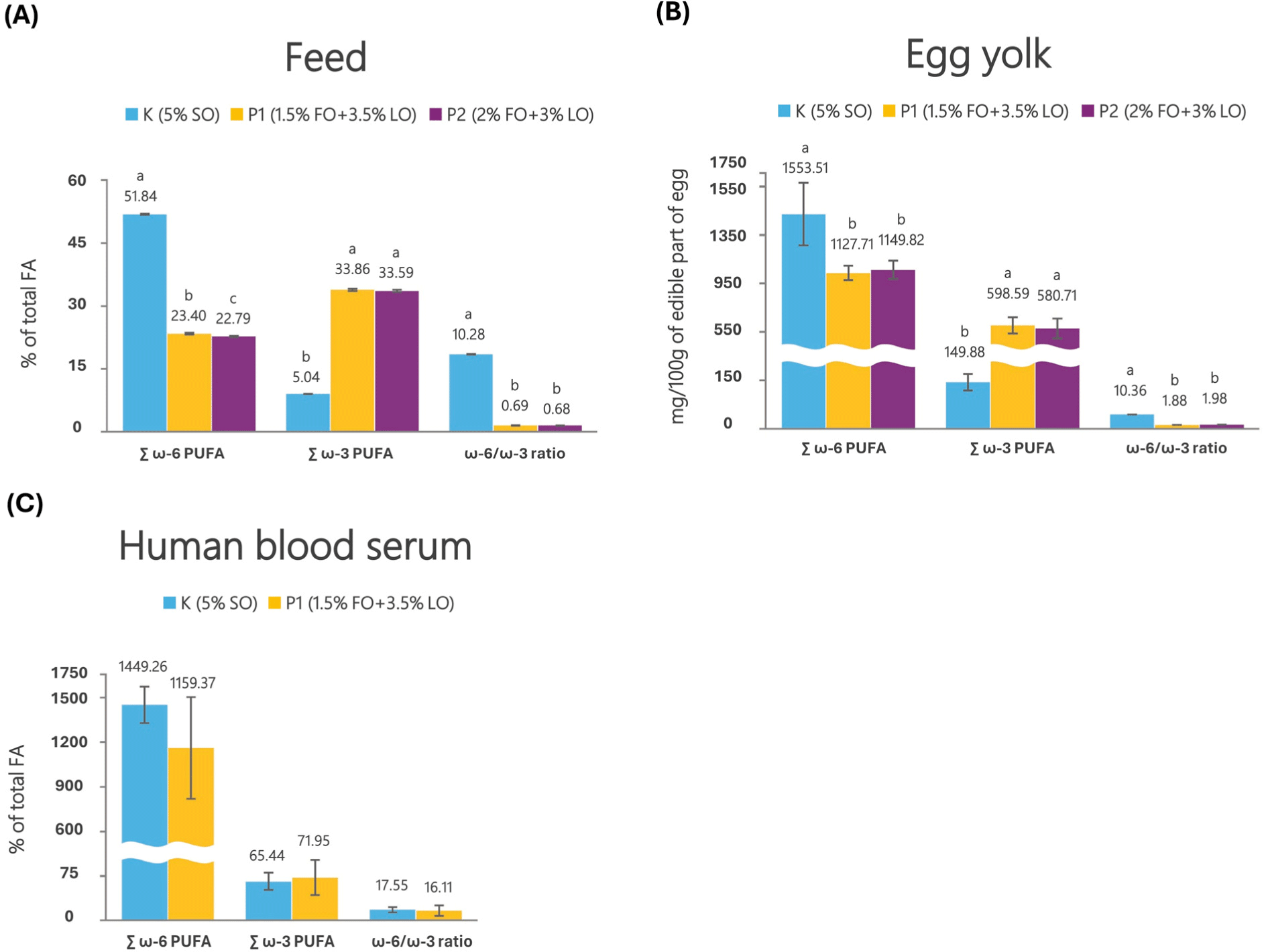
Pigs are ideal for producing meat fortified with ω-3 PUFAs by regulating dietary FA. In addition, pork is one of the most consumed meats, but it has a high level of saturated FA, so changes in the FA profile are necessary to improve its health properties. ω-3 stearidonic acid (SDA; C18:4 ω-3) is in a more advanced position than ω-3 ALA in the biosynthetic pathway. Therefore, SDA is converted to EPA and DHA more efficiently than ALA. Echium plantagineum is a good SDA source and grows abundantly in pastures. Recently, 60 crossbred gilts (Landrace×Large White) were fed palm oil (control), soya oil, linseed oil, fish oil, and Echium oil, respectively, and the fatty acid composition and ω-6/ω-3 ratio of M. longissimus thoracis were investigated (Fig. 10; van Wyngaard et al., 2023). ω-3 PUFAs were highest in the following order: fish oil 3.19%, linseed oil 2.52%, Echium oil 2.37%, soya oil 1.24%, and palm oil (control) 0.98%. The ω-6/ω-3 ratios were low in the following order: fish oil 4.05, Echium oil 4.93 and linseed oil 5.55, soya oil 12.43, and palm oil (control) 12.37. Dietary supplementation with fish oil, linseed oil, and Echium oil increased the ω-3 PUFAs content and decreased the ω-6/ω-3 ratio in M. longissimus dorsi muscle. The accumulation of long chain n-3 PUFAs (EPA+DPA+DHA) was in the following order: fish oil with high DHA and EPA content, Echium oil with high SDA content, linseed oil with high ALA content, and soya oil and palm oil (control) with high LA content. Echium oil supplementation did not increase ω-3 PUFA content to the same level as fish oil. However, when comparing Echium oil with linseed, soya, and palm oil, it improved long chain ω-3 PUFAs content in muscle without any adverse effects on meat quality. Along with E. plantagineum, seed oils of Buglossoides arvensis and Ribes sp. are also widely studied as sources of SDA to increase EPA and DHA status in animal foods. When Large White pigs were supplemented with 8% extruded flaxseed, which is a rich source of ALA, the level of ω-3 PUFAs increased approximately 9-fold, and the ω-6/ω-3 ratio decreased significantly from 20 to 2.5 (Tognocchi et al., 2023). In the fat-rich back fat, bacon, and ham fatty parts, the accumulation level of ω-3 PUFAs was higher than the threshold set by the EU to obtain the label “Source of ω-3 fatty acids”. These results showed that diet supplementation with 8% extruded linseed improved the nutraceutical quality of pork meat. Perilla is a rich source of ω-3 PUFAs, especially ALA, used in oil production.
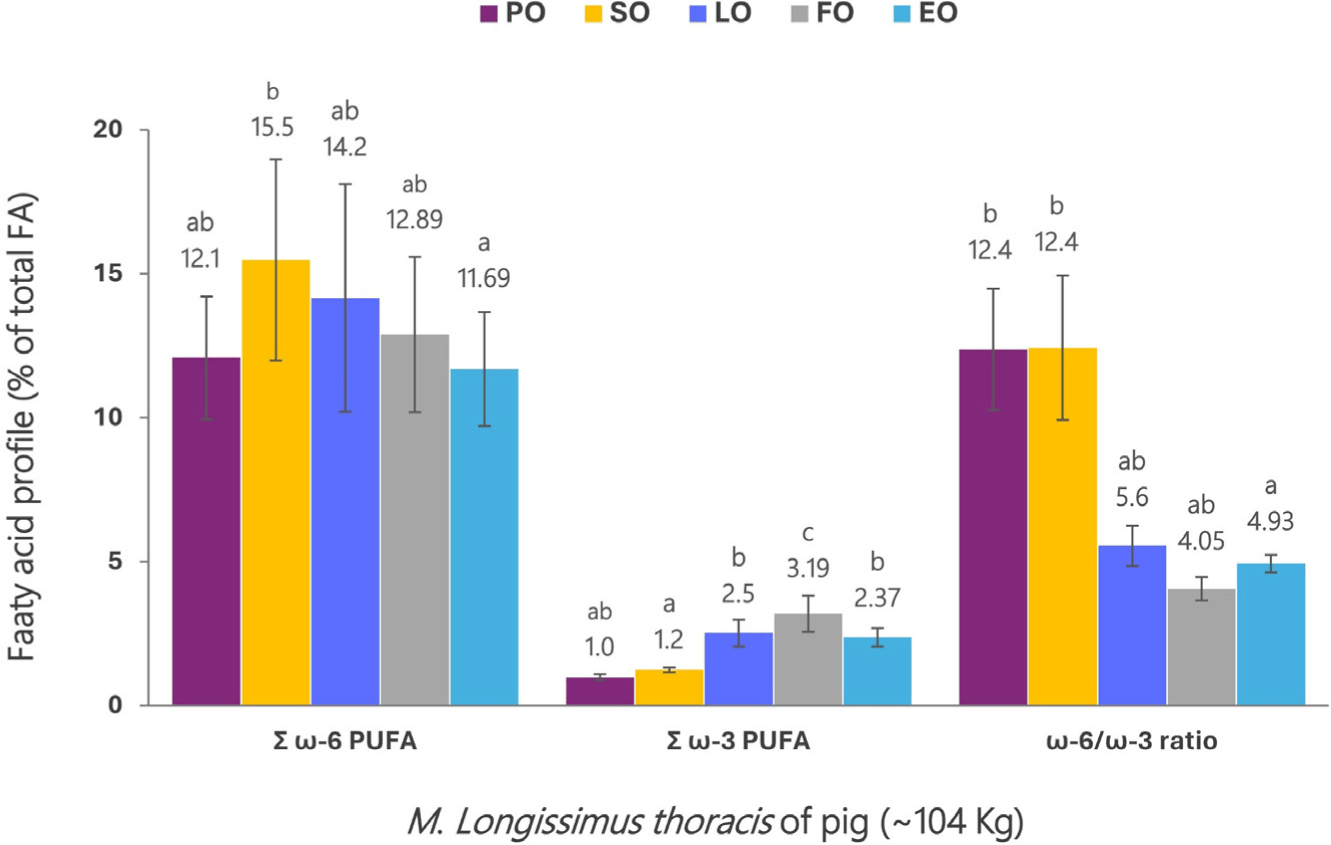
Perilla oil is composed of 90.60% total unsaturated fatty acids (UFAs), 17.90% MUFAs, and 72.70% PUFAs, and in particular, 55%–64% of PUFAs are ALA. Oil is extracted from perilla seeds using the screw pressing method and used commercially. This process produces perilla cake containing 9% to 10% fat as a by-product (Souphannavong et al., 2021). Supplementation of 2.5% perilla cake to the finishing crossbred pig diet increased intramuscular fat, marbling score, ALA, and decreased ω6/ω3 ratio in the longissimus thoracic et lumborum muscle. Supplementation of 2.5% perilla cake to the finishing crossbred pig diet changed the fatty acid composition required for healthy meat (Sringarm et al., 2022).
As consumer interest in grass-fed animal foods increases, differences in beneficial bioactive compounds, such as ω-3 PUFAs, micronutrients, and phytochemicals, between grass-fed and grain-fed animals are becoming more important. Over the past 30 years, research has shown that grass-based feeds can significantly improve beef’s ω-3 PUFAs composition and antioxidant content, with various effects on overall taste. Grass-fed animals have improved levels of the ω-3 PUFAs, conjugated linoleic acid (CLA), as well as antioxidants glutathione and superoxide dismutase activity compared to grain-fed animals (Daley et al., 2010). In Red Angus steers, grass-finished beef contained more ω-3 PUFAs and less ω-6 PUFAs than conventional grain-finished beef (Fig. 11; Krusinski et al., 2023). The experimental grass diet included diverse pasture (meadow fescue, clover, timothy grass, alfalfa, birds foot trefoil, chicory, orchard grass, and dandelion), and the experimental grain diet included a total mixed ration (TMR, orchard grass hay, corn, and pellets). The experimental grass diet contained 61.62% of ω-3 PUFAs, 14.09% of ω-6 PUFAs, and ω-6/ω-3 ratio of 0.24 in TFAs. On the other hand, the experimental grain diet contained 4.29% of ω-3 PUFAs, 56.12% of ω-6 PUFAs, and a ω-6/ω-3 ratio of 15.12. Compared to the grain diet, the grass diet had more than 14 times higher ω-3 PUFAs, while the ω-6 PUFAs were 4 times lower and the ω-6/ω-3 ratio was 63 times lower. Grass-finished beef (ribeye) showed ω-3 PUFAs 46.03 mg/100g, ω-6 PUFAs 67.07 mg/100g and ω-6/ω-3 ratio 1.65, while grain-finished beef had ω-3 PUFAs 10.13 mg/100g, ω-6 PUFAs 100.32 mg/100g and ω-6/ω-3 ratio 8.39. Compared to grain-finished beef, ω-3 PUFAs in grass-finished beef increased by 4.5-fold, and ω-6 PUFAs and ω-6/ω-3 ratio decreased by 1.5-times and 5.1-times, respectively. In particular, grass-finished beef decreased the sum of FAs while increasing ALA, EPA, and DPA. Grasses generally contain higher levels of PUFAs (especially ω-3) when compared to grains. The higher concentration of ω-3 PUFAs in grasses is due to the accumulation of FAs in the leaf tissue of fresh pasture, and ω-3 PUFAs generally account for 50%–75% of FA composition (Alothman et al., 2019). Additionally, pasture-finishing (i.e., grass-fed) bison reduced ω-6/ω-3 ratios (<3.2), improved metabolic health, and accumulated potential health-promoting compounds compared to pen-finished bison on concentrates (van Vliet et al., 2023). Grass-finished beef contains more ω-3 PUFAs and a lower ω-6/ω-3 ratio than grain-finished beef. It contains more beneficial bioactive compounds such as micronutrients and phytochemicals, making it suitable for separating beef based on finishing feeds. It shows the possibility of production strategy.
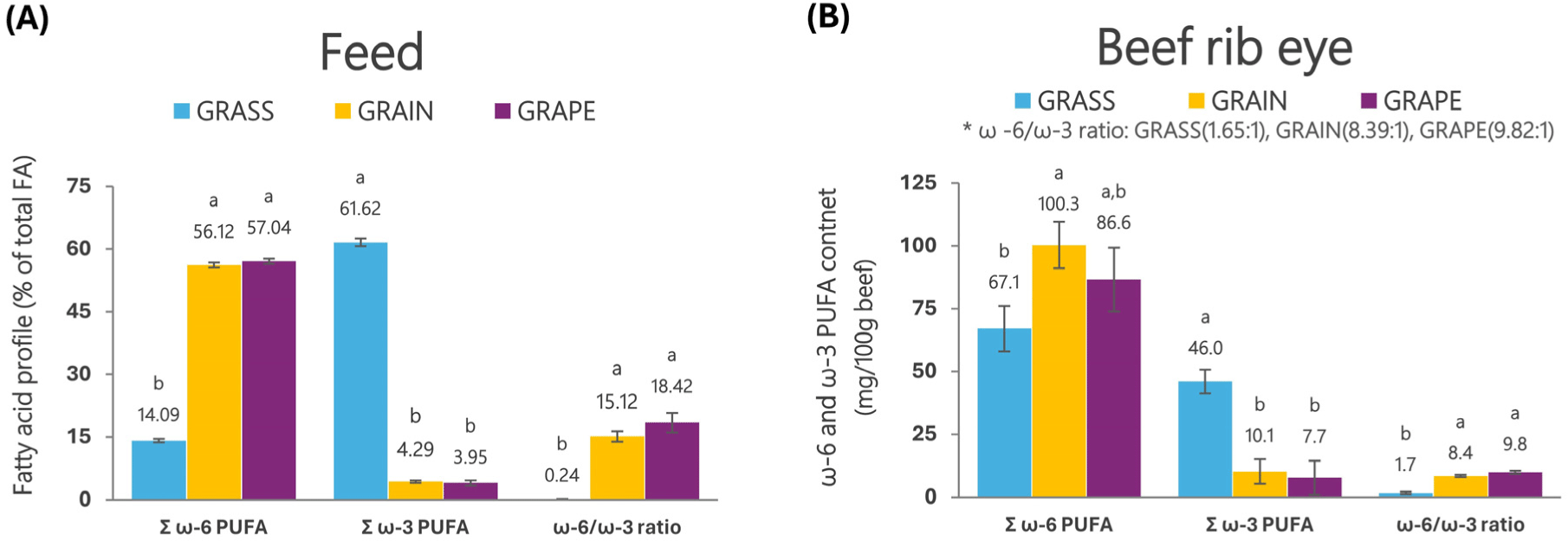
To increase the content of ω-3 PUFAs in cows’ milk, linseed oil, fish oil, and microalgae are used as dietary supplements. Linseed oil is mostly composed of UFAs, and contains 15.19% and 54.21% of LA and ALA, respectively. When this linseed oil enters the rumen, loss of ω-3 PUFAs occurs due to an extensive biohydrogenation process. Therefore, dietary supplementation with rumen bypass microencapsulated linseed oil can prevent the loss of ω-3 PUFAs and increase the ω-3 PUFAs content in milk. The milk of the control group fed TMR from Holstein dairy cows contained 9.24 mg/100 g of ω-3 PUFAs, 63.40 mg/100 g of ω-6 PUFAs, and ω-6/ω-3 ratio of 6.89. However, milk from the treatment group receiving dietary supplementation with 2% microencapsulated linseed oil contained 58.58 mg/100 g of ω-3 PUFAs, 17.77 mg/100 g of ω-6 PUFAs, and 3.48 of ω-6/ω-3 ratio. Dietary supplementation of 2% microencapsulated linseed oil increased the ω-3 PUFAs content and lowered the ω-6/ω-3 ratio in the milk of dairy cows, and confirmed that rumen bypass microencapsulated fatty acids were safely transferred from the rumen to the small intestine (Fig. 12; Kim et al., 2020).
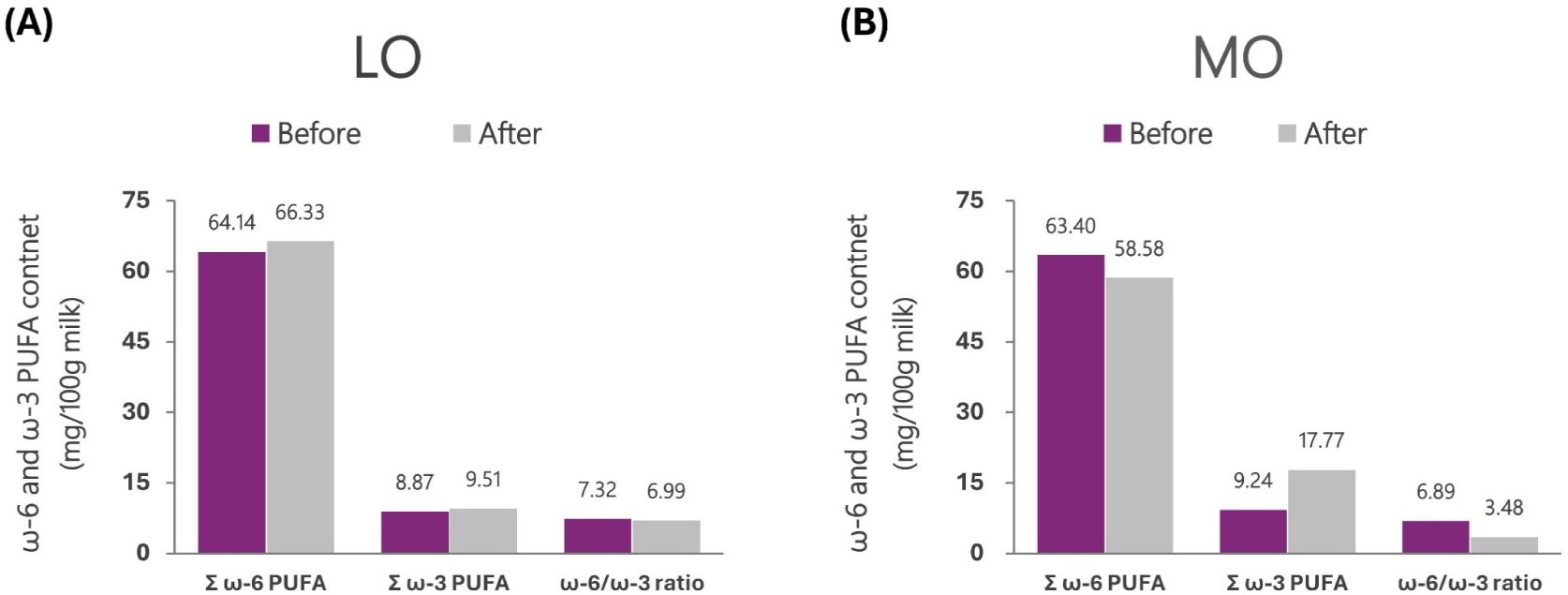
In a study to enhance the nutritional value of goat milk and raw cheese, the control diet was supplemented with 1.55%/DM palm oil and the two experimental diets were supplemented with 3.88%/DM flaxseed flakes and 2.64%/DM fish oil (Moya et al., 2023). The fatty acid profile of milk fed a control diet (palm oil 1.55%) showed 20.59 mg/100 g of ω-3 PUFAs, 190.61 mg/100 g of ω-6 PUFAs and 9.26 of ω-6/ω-3 ratio. The fatty acid profile of milk fed with 3.88%/DM flaked linseed diet showed 62.60 mg/100 g of ω-3 PUFAs, 216.14 mg/100 g of ω-6 PUFAs, and 3.45 of ω-6/ω-3 ratio. The fatty acid profile of milk fed with 2.64%/DM fish oil showed 45.27 mg/100 g of ω-3 PUFAs, 208.24 mg/100 g of ω-6 PUFAs and 4.59 of ω-6/ω-3 ratio. Compared to the control diet, the 3.88%/DM flaked linseed diet increased ω-3 PUFAs by 3 times, and the ω-6/ω-3 ratio decreased by 2.7 times. These results were the same for fresh goat cheese. The flaked linseed-supplemented diet improved the nutritional value of milk due to a decrease in SFAs and an increase in PUFAs and CLA. Dietary supplementation with flaked linseed or fish oil produced goats’ milk and cheese with higher nutritional quality without affecting the sensory profile of the corresponding products obtained from animals fed routine diets.
According to the OECD/FAO report, the world’s per capita consumption of milk and dairy products in 2015 was 111.3 kg and is expected to increase by about 12.5% by 2025 (OECD and FAO, 2017). Due to this, much research has focused on enriching ω-3 PUFAs in milk, and its processed products (mostly from cows and sheep) consumed by humans (OECD and FAO, 2017).
Because ω-6 and ω-3 PUFAs are used competitively by desaturase and elongase enzymes that produce inflammatory and anti-inflammatory eicosanoids, a balanced intake of ω-6 and ω-3 PUFAs is necessary to suppress chronic diseases and maintain good health. The recommended value of EPA and DHA for the European adult population is 250 to 500 mg/d, and the recommended dietary ratio of ω-6/ω-3 is 1:1–2:1 (Avallone et al., 2019). However, the ratio of ω-6/ω-3 in typical Western diets is 15/1 to 16.7/1 (Simopoulos, 2006), and the amount of DHA contained in Western foods is approximately 100 mg/day (Cardoso et al., 2016). In human hepatoma cells, EPA and DHA conversion rates are highest when ω-6/ω-3 is at a 1:1 ratio, resulting in 17% and 0.7% rates, respectively (Harnack et al., 2009). Therefore, balanced intake of ω-6 and ω-3 PUFAs and direct DHA supplementation are the most effective ways to increase body DHA levels.
ω-6 Polyunsaturated Fatty Acids and Gut Microbiota
Gut microbes exist throughout the mammalian gastrointestinal tract and come into contact with all ingested dietary fats. Therefore, enzymes in gut microbes serve as a second liver to break down, transform, and detoxify dietary components that can have beneficial and detrimental impacts on host health (Brown et al., 2023). Just as the function of liver enzymes is to digest dietary and exogenous fats, gut microbial enzymes play a similar role in the gut. However, most functional by-products of gut microbial enzymes are still unknown. Dietary PUFAs may also be saturated by common gut microbial enzymes, limiting the number of double bonds and oxidative potential. Gut microbiomes containing Bifidobacterium and Lactobacillus species produce PUFA-derived intermediate metabolites, and PUFA-derived bacterial intermediate metabolites produced in the intestine exhibit anti-obesity and anti-inflammatory effects (Kishino et al., 2013). Gut microbes convert ω-6 LA into 10-hydroxy-cis-12-octadecenoic (HYA), reducing adipose inflammation and obesity caused by excessive dietary ω-6 LA and AA cascade.
Additionally, HYA promotes the secretion of glucagon-like peptide-1 (GLP-1) by activating G protein-coupled receptors GPR40 and GPR120. GLP-1 improves glucose homeostasis and suppresses appetite. HYA promotes intestinal peristalsis through EP3 (E-prostanoid 3 receptor) and inhibits lipid absorption (Fig. 13; Miyamoto et al., 2019).
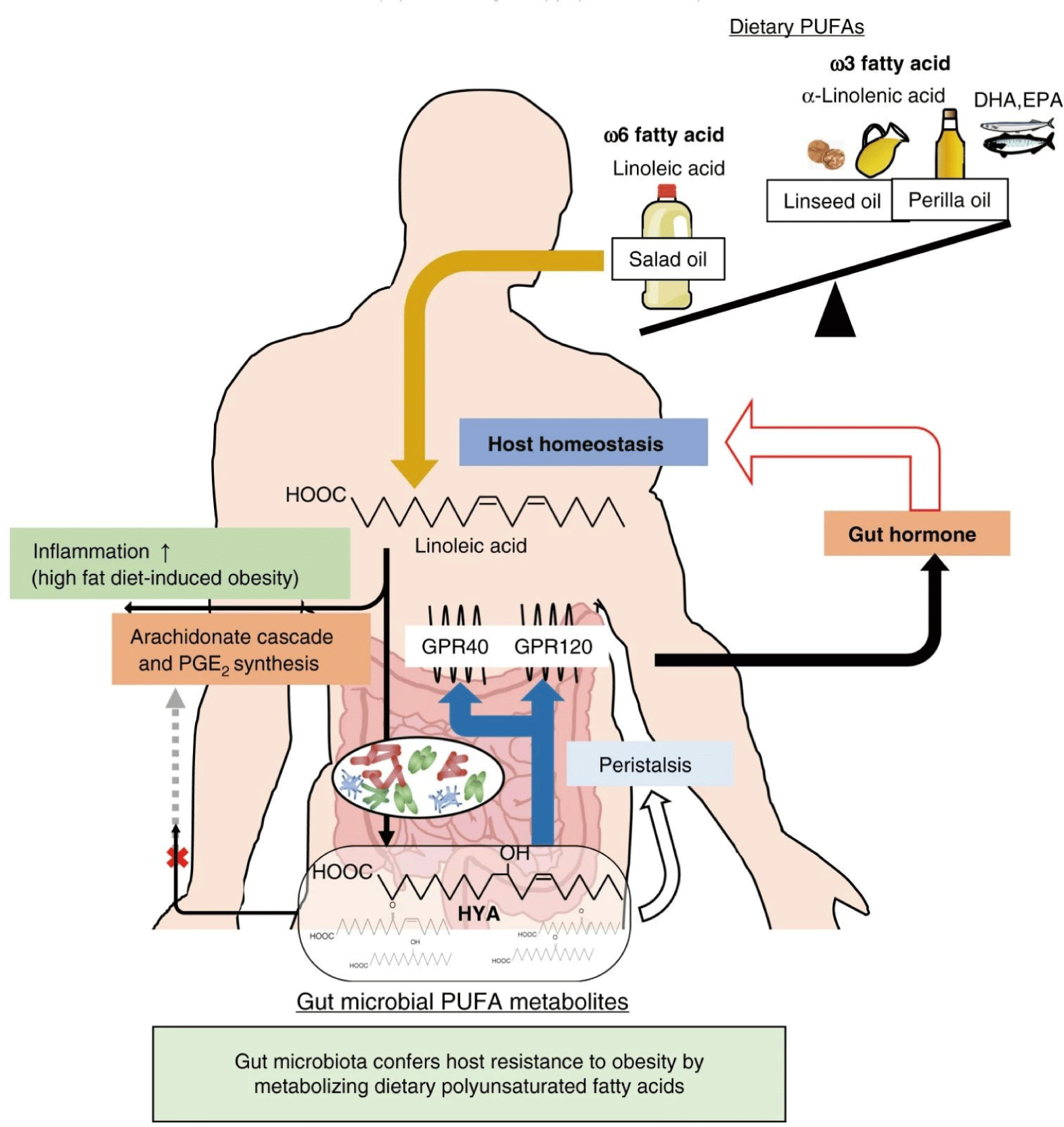
In db/db mice, EPA and DHA supplementation decreased the amount of LPS-containing Enterobacteriaceae. It increased the number of beneficial Bifidobacterium, Lactobacillus, and short-chain fatty acid (SCFA)-producing species (Zhuang et al., 2021). The genus Lactobacillus is a facultatively anaerobic bacteria abundant in the small intestine, especially the ileum. This is consistent with the fact that fats are absorbed in the small intestine and that Lactobacillus-derived fatty acids HYA are abundant in the ileum. ω-6 LA supplementation diet causes significant expansion of the intestinal Lactobacillus genus. Among the Lactobacillus genus, Lactobacillus salivarius has the high activity of a critical LA-metabolizing enzyme conjugated linoleic acid-oleate hydratase (Cla-hy), which converts LA into HYA, and LA induces the expression of Cla-hy gene. Additionally, Lactobacillus plantarum possesses CLA-HY, CLA short-chain dehydrogenase (CLA-DH), and CLA acetoacetate decarboxylase (CLA-DC) activities. These enzymes synthesize HYA and CLAs from LAs and have potential physiological activities such as anti-inflammatory, anti-CVD, and anti-diabetes (Liu et al., 2021). These research results provide a central mechanism explaining the interrelationship between commensal bacteria and the host for maintaining energy homeostasis. They can be used to develop customized functional probiotics and foods to prevent metabolic disorders such as obesity, CVDs, and type 2 diabetes.
Conclusion
EPA and DHA are well known to prevent numerous metabolic disorders. Due to the increase in chronic diseases, consumer interest and demand for natural dietary sources of ω-3 PUFAs are rapidly increasing. ω-6 PUFAs and ω-3 PUFAs have beneficial health effects and antagonistic (opposing) metabolic functions in the body. ω-6 AA accounts for up to 25% of the fatty acids in phospholipids of muscles, brain, liver, platelets, and immune cells (Calder, 2007). PLA2 releases AA from the membrane into the cell cytosol (Korotkova and Lundberg, 2014). In “resting” conditions, eicosanoid production is low and the metabolites produced play a role in maintaining homeostasis by regulating physiological processes. However, AA is released in the presence of inflammatory stimuli and eicosanoid formation increases. ω-3 EPA and DHA have various anti-inflammatory effects and reduce AA-derived eicosanoid production. It also competes with AA to form of cell membrane phospholipids and reduces the release of AA from membranes (Calder, 2015). EPA and DHA play various roles in maintaining body homeostasis and protecting against several chronic and metabolic diseases, including cardiovascular, inflammation, obesity, and diabetes.
ω-6 PUFAs greatly exceed the amount of ω-3 PUFAs in most Western diets. Modern diet-related chronic diseases are rising due to the increasing unbalanced consumption of ω-6 PUFAs rather than ω-3 PUFAs. The essential fatty acids of LA and ALA compete with the same set of enzymes in the elongation and desaturation processes that are converted to longer PUFAs. When ω-6 LA is present at a higher ratio than ω-3 ALA, LA is converted to AA, so most cellular phospholipids become AA. In oxidative metabolism, AA produces many potent pro-inflammatory eicosanoids and contributes to the formation of thrombus and atheroma, allergic reaction and inflammatory disorders, and a hyperactive endo-cannabinoid system that affects appetite and food intake, increasing weight gain and obesity. On the other hand, ω-3 PUFAs, such as EPA and DHA, have anti-inflammatory, anti-aggregatory, vasodilation, and bronchodilation effects, resolving inflammation and reducing the risk of cancer and CVDs by changing the function of vascular and carcinogen biomarkers. Therefore, considering these antagonistic effects of ω-3 and ω-6 PUFAs, balancing the LA/ALA ratio is important in regulating body homeostasis.
Among plant sources, seed oils of Salvia hispanica, Linum usitatissimum, Lepidium sativum, E. plantagineum, B. arvensis, and Ribes sp. are widely studied as sources of ALA and SDA. Additionally, oils from microalgae and thraustochytrids can directly supply EPA and DHA. Therefore, these plant sources are used for the commercial production of EPA and DHA (Saini et al., 2021).
Monogastric animals, such as pigs and chickens, are the ideal for producing fortified meat and eggs with altered FA profiles by controlling dietary FA. Supplementing animal feed with oils high in ω-3 fatty acids and antioxidants that limit lipoperoxidation has an antioxidant effect on feed lipids. It also produces ω-3 PUFA enriched meat and has higher oxidative stability. These results serve as the basis for producing meats with desirable FA profiles and oxidative stability by using ω-3 sources and antioxidant additives in feed mixtures (Fig. 14).
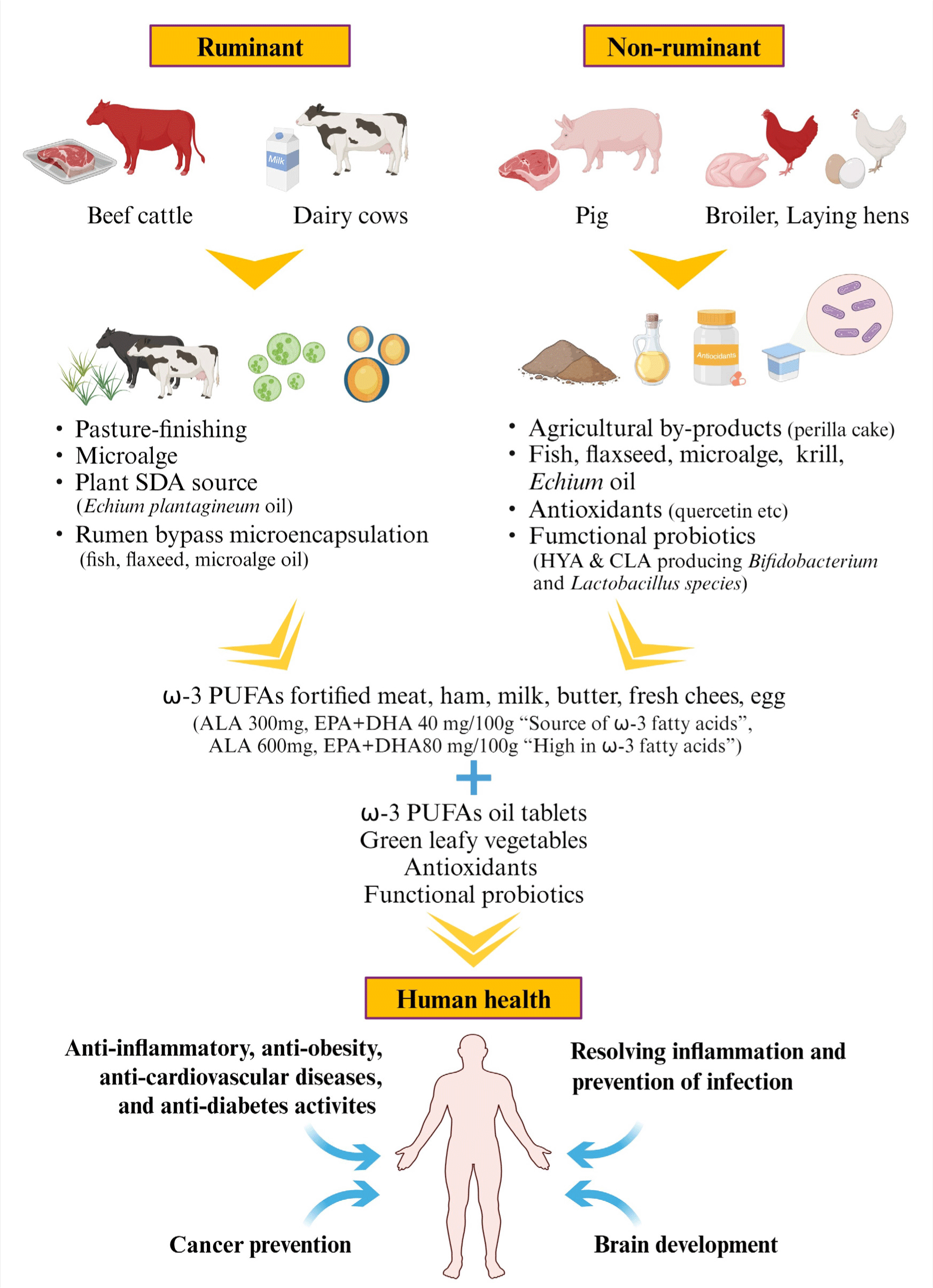
Cattle management practices should be considered when making nutritional claims. Grass-finished beef produced in agroecological ways matches the preferences of consumers concerned about its impact on well-being and environmental impact. Grass-finished beef is an important alternative that contributes to food sustainability goals by providing higher amounts of potentially beneficial nutrients. Compared to grain-fed beef, grass-fed beef has higher ω-3 PUFAs and CLA and lower ω-6 PUFAs and cholesterol-raising SFAs. This protects against several metabolic diseases, including obesity, diabetes, cancer, and CVD. Therefore, beef’s ω-6/ω-3 ratio, total ω-3 PUFAs, micronutrients, and phytochemicals are essential compounds in determining the finishing diet of cattle. Grass-finished beef, which contains high amounts of beneficial bioactive compounds, is beneficial to human health.
Supplementing the diets of lactating dairy cows with canola oil, linseed oil, and rumen-protected microencapsulated fatty acids can increase the concentration of ω-3 PUFAs, CLA, and OA, producing more nutritionally enhanced milk. Rumen-bypass microencapsulated ω-3 fatty acids safely improve the pass rate of ω-3 fatty acids from the rumen to the intestine and do not negatively influence ruminal fermentation in dairy cows. This rumen-protected microencapsulation approach of ω-3 fatty acids sources represents a strategy for producing meat and milk enriched with ω-3 fatty acids for ruminants. To produce meat, milk, and cheese high in ω-3 PUFAs from ruminant livestock, including lactating dairy cows and goats, dietary supplementation with ω-3 sources or pasture finishing and rumen-protected microencapsulated fatty acids can be selected. This choice benefits human health by improving animal metabolic health and nutritional compounds. Therefore, much research is needed in the future to link the impact of various production systems on the environment (soils, plants, animals) and human health.
A higher LA/ALA ratio than the high amount of fats included in the diet is the primary determinant of disease aggravation. A lower LA/ALA ratio has a prebiotic effect that suppresses abnormal expansion of Proteobacteria and Escherichia-Shigella. Additionally, the interaction between gut microbiota and host energy metabolism through dietary ω-6 PUFA-derived bacterial intermediate metabolites provides a method to prevent metabolic disorders by targeting gut microbial metabolites.
A high ω-6/ω-3 ratio is associated with overweight and obesity, while a balanced ratio reduces obesity and weight gain. Therefore, the intake of ω-3 PUFAs should be increased while reducing ω-6 PUFAs in human diet and animal feed. Additionally, it is necessary to make every effort to increase the intake of probiotics, including lactic acid bacteria, which can convert ω-6 fatty acids into functional metabolites.