Introduction
The quality and shelf life of meat products during storage are decreased by product deterioration. One of the main factors reducing the quality and shelf life of muscle-based foods is oxidative changes including lipid and protein oxidation (Dave and Ghaly, 2011; Lund et al., 2011). Meatballs contain minced meat, in which oxidation occurs more rapidly than in intact meat because the surface area in contact with air is increased by grinding (Naveena et al., 2008). The detrimental effects of lipid oxidation include the loss of fatty acids and reduced flavor, leading to changes in sensory properties (Maqsood et al., 2014). Protein oxidation affects the quality of meat products by altering the tenderness, water-holding capacity, and nutritional quality, as well as induces carbonyl formation, reduces sulfhydryl content, and alters amino acid structures. Proteins may be modified by compounds formed during lipid oxidation, thereby reducing protein function. Thus, protein oxidation has attracted attention in recent years (Bekhit et al., 2013; Lund et al., 2011).
Natural and synthetic antioxidants have been widely examined to reduce oxidation in meat products. However, synthetic antioxidants such as butylated hydroxy anisole and butylated hydroxy toluene can exert carcinogenic effects in food (Ito et al., 1986). Therefore, researchers have focused on safe and natural antioxidants rather than synthetic antioxidants. Recently, many studies were conducted to evaluate natural antioxidants such as fruits, vegetables, and herbs including rosemary extract (Lund et al., 2007), Nitraria retusa (Mariem et al., 2014), and pomegranate peel (Kanatt et al., 2005).
Recently, Allium hookeri was successfully cultivated in South Korea. Allium species are traditionally used for medicinal purposes in Korean folk medicines and show antioxidant and anti-inflammatory effects (Nishimura et al., 2006). Allium species contain sulfur compounds such as methiin, alliin, allicin, isoalliin, and propiin (Rhyu and Park, 2013). Cho et al. reported that 1 h water extracts of Allium hookeri root (AHR) effectively reduced the lipid oxidation rates of meat patties during storage.
These antioxidant characteristics of sulfur compounds in AHR may affect the shelf life of meat products. Therefore, the objective of this study was to determine the effects of AHR addition on the texture properties, color, and oxidative stability of lipids and proteins in meatballs.
Materials and Methods
AHRs were purchased (Samchaenara Co., Pyeongtaek, Korea) and freeze-dried (Dongil Cold Storage and Foods Co., Icheon, Korea). AHR contained 8,580.16±28.40 mg/kg of sulfur compounds (National Instrumentation Center for Environmental Management, Seoul, Korea). Longissimus dorsi muscle and pork back fat were purchased to prepare meatballs from a local slaughterhouse in Seoul, Korea.
Meatballs were manufactured using longissimus dorsi (75%), back fat (25%), and NaCl (1.5%). All ingredients were mixed in a bowl mixer for 7 min and divided into 4 groups. AHR was added to two groups at concentrations of 0.5% (w/w) and 1% (w/w). The third group contained ascorbic acid at 0.05% (w/w) as a reference, and the final group did not contain the antioxidant. Meatballs (30±2 g) were formed into a round shape by hand and boiled at 80℃ for 30 min. A total of three batches were made and six meatballs were placed in plastic trays (15×15×4 cm) corresponding to 0 d, 4 d, 8 d, and 14 d per batch, covered with polyvinyl chloride wrap (O2 transmission: 0.20 mL/min), and stored in the dark at 4℃ for 14 days. Samples were taken at 0 d, 4 d, 8 d, and 14 d of storage.
Two-gram samples were homogenized with 18 mL of distilled water by using a bag mixer 400 (Interscience Co., St Nom la Bretêche, France) for 1 min. The pH values of homogenized samples were measured with a pH meter (F-71G, Horiba Co., Kyoto, Japan).
The color was expressed according to the Commission International de l’Eclairage system and reported as CLE L* (lightness), CIE a* (redness), and CIE b* (yellowness). Color was measured with a colorimeter (Chromameter Minolta Co., Osaka, Japan) calibrated with a white standard plate (CIE L*: +97.30, CIE a*: –0.09, CIE b*: +1.94) using an 8 mm diameter measuring area and 50 mm diameter illumination area. Measurements were conducted in triplicate for each sample.
Texture profile analysis (TPA) was conducted using a TA-XT2i (Stable Micro Systems, Godalming, UK). The meatballs were cut in a half, and two pieces of 1×1×1 cm were collected and measured. The texture analyzer was used with the following settings: pre-test speed of 2.0 mm/s, test speed of 2.0 mm/s, post-test speed of 8.0 mm/s, time of 2.0 s, distance of 7.0 mm, and trigger force of 5 g. Values for hardness (kg), springiness, cohesiveness, gumminess (kg), and chewiness (kg) were determined as described by Bourne (1978).
Volatile basic nitrogen (VBN) was evaluated to determine the extent of protein deterioration. Three grams of sample were homogenized with 9 mL distilled water. The mixture was filtered into a 50 mL messcylinder, and distilled water was added to 50 mL, and 1 mL extract was converted into Conway’s unit. VBN was determined using a modified micro diffusion assay as described by Pearson (1968).
Lipid oxidation was determined according to the TBARS method as described by Buege and Aust (1978). Firstly, 3 g of sample was homogenized at 3,220×g for 30 s in 9 mL distilled water and 60 μL 6% (w/v) butylated hydroxytoluene in ethanol. Next, 2 mL of the homogenate was reacted with 4 mL of TBA reagent (20 mM 2-thiobarbituric acid in 15% trichloroacetic acid [TCA] solution). The mixture was heated for 15 min in an 80℃ water bath. After cooling in ice water, the samples were centrifuged at 2,000×g for 10 min. The supernatant was filtered through Whatman filter paper No. 1 and the absorbance of the samples was evaluated at 532 nm (A532) with a spectrophotometer (Optizen 2120UV, Mecasys, Seoul, Korea). The malondialdehyde (MDA) concentration was converted to a TBARS value using the equation shown below and molecular extinction coefficient of 1.56×105 M–1cm–1.
Protein carbonyl content was estimated as described by Levine (1994) with some modifications. A 1 g sample was homogenized with 10 mL of 20 mM phosphate buffer (pH 6.5) containing 0.6 M NaCl. Four aliquots (0.2 mL) were collected, and 1 mL of ice-cold 10% (w/v) TCA was added, followed by incubation for 15 min in an ice bath. After centrifugation at 2,000×g for 30 min, the supernatant was removed; 1 mL of ice-cold 10% (w/v) TCA was added, and the sample was incubated for 15 min in an ice bath. The sample was centrifuged at 2,000×g for 30 min, and the supernatant was separated into two aliquots with 0.5 mL of 10 mM 2,4-dinitrophenylhydrazine solution and two aliquots containing 5.0 mL of 2.0 M hydrochloride (blank). After incubation in a shaker for 1 h (in the dark, 150 rpm), 0.5 mL of ice-cold 20% (w/v) TCA was added, and then the sample was vortexed and placed in an ice bath for 15 min. After centrifugation at 2,000×g for 20 min, the supernatant was removed, and the pellets were washed three times with 1 mL ethanol/ethyl (1:1, v/v) acetate solution, vortexed, and centrifuged at 2,000×g for 20 min. Excess solvent was evaporated for 15 min under a hood. One milliliter of 6 M guanidine hydrochloride in 20 mM phosphate buffer (pH 6.5) was added, and the sample was incubated in a shaker for 30 min (in the dark, 150 rpm). After the sample was centrifuged at 9,500×g for 10 min, protein concentration was measured by measuring the absorbance at 280 nm using a bovine serum albumin standard curve. Carbonyl content was measured at an absorbance of 370 nm using a molar extinction coefficient of 21,000 M–1cm–1 (nmol/mg protein).
The total sulfhydryl concentration was determined using 5,5'-dithin-bis-2-nitrbenzoic acid (DTNB) as described by Vossen and De Smet (2015). One gram of sample was homogenized at 3,220×g with 20 mL of 5% (w/v) SDS buffer followed by 1 h of incubation in a water bath at 80℃. After cooling, the samples were filtered through Whatman paper No. 1 and 2 mL of 0.1 M Tris buffer and 0.5 mL of 10 mM DTNB in 0.1 M Tris buffer were added to 0.5 mL sample for a reaction time of exactly 30 min (in the dark). The blank contained a mixture of 0.25 mL of 5% (w/v) SDS in Tris buffer, 0.25 mL of 10 mM DTNB, and 1 mL of 0.1 M Tris buffer. Protein concentration was measured by the biuret test using bovine serum albumin as a standard. The absorbance of the sulfhydryl group was measured at 412 nm (A412), and protein concentration was detected at 550 nm.
where ε is molar extinction coefficient, 11,400 M–1cm–1.
Experiments were conducted three times, all parameters were measured in triplicate, and the standard deviation was reported. All data were analyzed using SPSS Ver. 24.0 (SPSS, Inc., Chicago, IL, USA). One-way analysis of variance and Duncan’s multiple range tests were used to determine the differences among treatments (p<0.05).
Results and Discussion
Fig. 1 shows the change in pH of the meatballs during storage. The pH values were significantly different among the treatment and control groups during storage (p<0.05). The initial pH values were 5.97–5.99; those of T2 and T3 were significantly higher than that of T1 at day 0 (p<0.05). According to Choi et al. (2010), the high pH of frankfurters is related to the minerals in the additives. Additionally, Park and Yoon (2014) reported that Korean AHR contains minerals such as Ca, Cu, Fe, K, Mg, Mn, Mo, Na, P, and Z (total 1,371.64 mg/100 g). The pH of T1, T2, and T3 decreased significantly after 4 d of storage (p<0.05). This is consistent with the results of Shin et al. (2017), who showed that pH decreases because of the activity of lactic acid bacteria over the storage period. However, after 8 d, the pH increased. The increase in pH occurred because of the production of basic amines by microorganisms (Masrniyom et al., 2002). pH changes because of various factors such as the accumulation of basic molecules produced by microbial growth (Masniyom et al., 2002), activity of lactic acid bacteria (Shin et al., 2017), and mineral content of additives (Choi et al., 2010). However, over the total storage period, the pH of the control group decreased by 0.1 and that of T1, T2, and T3 showed a difference of ±0.08 in the initial and final values, which was not significant. Therefore, AHR did not significantly affect the pH during the storage period.
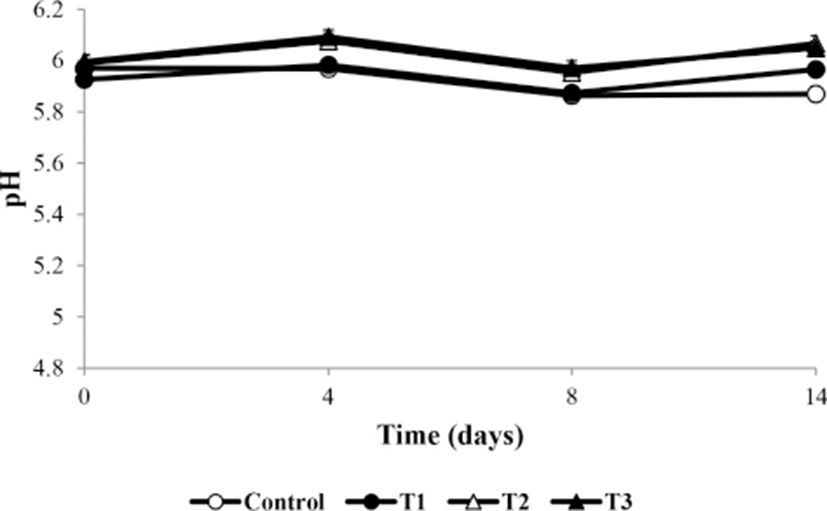
Changes in the lightness, redness, and yellowness of meatballs during storage are shown in Table 1. Initially, the CIE L* and CIE a* values were significantly lower in T2 and T3 than in the control (p<0.05). Similar to the results obtained by Cho et al. (2015), pork patty containing AHR extract initially showed low CIE L* and CIE a* values. This parameter is affected by the pigment components of AHR. The CIE L* and CIE b* values increased with increasing storage time, except for in T1 (p<0.05). The CIE a* values of meatballs in all groups tended to decrease with increasing storage time, whereas those in T1 did not significantly differ during storage (p>0.05). The decreases in the CIE a* values of meatballs may have occurred because of lipid oxidation and microorganisms. In aerobic environments, microorganisms responsible for greening generate hydrogen peroxide and oxidize myoglobin to cholemyoglobin (Mills et al., 2014). The CIE b* values of T2 and T3 were significantly increased during the storage period (p<0.05). The CIE b* value increased following heat treatment because of the Maillard reaction of the sugar and amino acid components of AHR. Terns et al. (2011) reported that both the CIE L* and CIE b* values increased during storage. These results indicate that addition of AHR changed the color characteristics of meatballs by increasing yellowness and decreasing redness.
Table 2 shows the changes in TPA in meatball during storage. The springiness, gumminess, cohesiveness, hardness, and chewiness values of T2 and T3 were lower than the initial values of the control. The initial low hardness value is softened by the dilution effect when non-meat ingredients are added to the meat protein system (Aleson-Carbonell et al., 2005). The total meat contents of meatball were 75% of longissimus dorsi and 25% of back fat. However, the nonmeat ingredient of T1 were 1.5% NaCl and 0.05% ascorbic acid, of T2 were 1.5% NaCl and 0.5% AHR, and of T3 were 1.5% NaCl and 1% AHR. Therefore, addition of non-meat ingredients (T1: 1.55%, T2: 2%, T3: 2.5%) resulted in low hardness because of dilution effects (over 100%). After 14 d, the gumminess, cohesiveness, hardness, and chewiness of meatballs in all groups decreased significantly (p<0.05). TPA values decreased during storage because of the proteolytic actions of microorganisms (Maqsood et al., 2012). Therefore, the physical properties of meatballs depend on the composition of the ingredients that make up the meatballs.
Fig. 2 shows the VBN value of meatballs during storage, which did not significantly differ from the initial VBN values (p>0.05). However, the VBN value of T3 was significantly lower than that of the control at 14 d (p<0.05). The VBN value, which is associated with endogenous enzymes and the activities of various spoilage microorganisms, is an index of meat deterioration (Cai et al., 2015). The VBN value generally increases because of the proliferation of proteolytic microorganisms and chemical changes during the storage period (Cuong and Chin, 2016). In the AHR component, when alliin is attacked by microorganisms, it is converted to volatile alkyl sulfinyl compounds, which have antibacterial abilities (Ariga and Seki, 2006; Rhyu and Park, 2013). Therefore, our results suggest that sulfur compounds such as alliin in AHR inhibited microbial growth and decreased the VBN values by preventing protein decomposition.
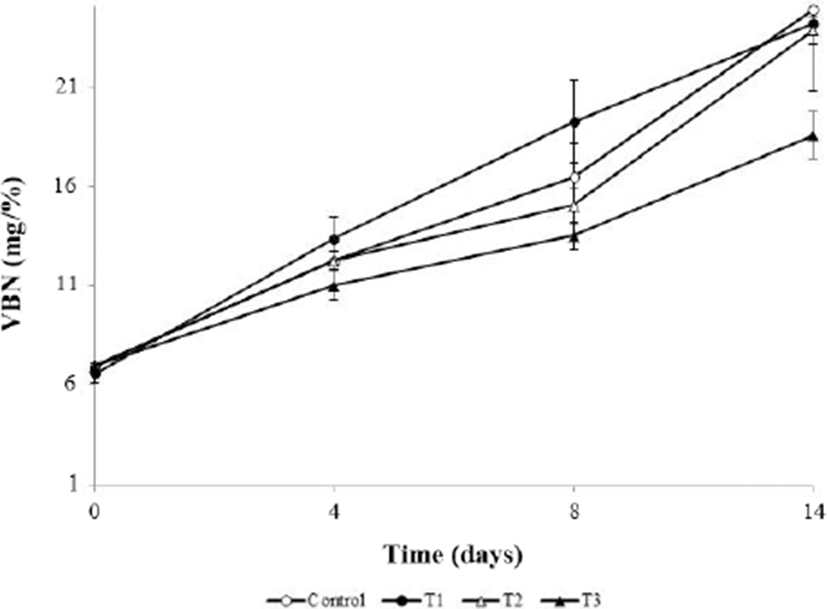
Fig. 3 shows the changes in TBARS values in meatballs during refrigerated storage for 14 days. TBARS values were significantly lower in T1, T2, and T3 than the initial value in the control (p<0.05). Cell membrane collapse mainly occurred following the heating process, leading to the release of pro-oxidants and acceleration of lipid oxidation in the meat (Gray and Pearson, 1987; Tichivangana and Morrissey, 1985). The TBARS value mainly indicates the MDA content, which is a secondary product of lipid oxidation and known to cause the deterioration of meat quality (Choe et al., 2017). TBARS values began increasing at 4 days, with T1 showing the highest values (p<0.05). At day 14, the TBARS values of T2 and T3 were significantly lower than that of the control (p<0.05) and T1 showed the highest values. According to Zhao and Jung (1995), TBARS values of ascorbic acid are increased when ascorbic acid reacts with a metallic ion to produce H2O2, which causes lipid peroxidation. Additionally, ascorbic acid did not effectively prevent lipid oxidation when used alone as an additive (Sánchez-Escalante et al., 2001). Previous studies demonstrated the high phenolic content in A. hookeri and antioxidant effects of A. hookeri powder and extracts on fresh meat products during storage (Cho et al., 2015; Song et al., 2014). The present findings indicate that the antioxidant effect of A. hookeri on cooked meat products is stable, thus extracts from this species can be used as a substitute for synthetic antioxidants.
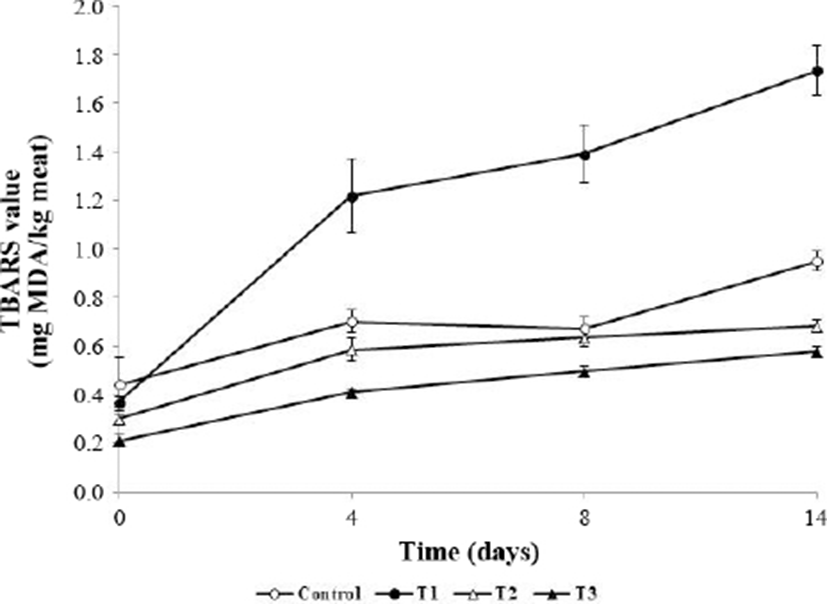
As shown in Fig. 4, protein carbonyls in meatballs were significantly affected by AHR addition and storage time (p<0.05). Initial carbonyl contents were 2.44, 2.91, 2.39, and 2.13 nmol/mg protein in the control, T1, T2, and T3 groups, respectively. Although the mean carbonyl content of the control was 2.66 nmol/mg, that of meatballs containing 1% (T3, w/w) AHR was 1.71 nmol/mg protein at 14 d. The increase in carbonyl content in all groups at 8 days resulted in oxidative reactions during refrigerated storage; carbonyl contents were significantly affected by storage time (p<0.05) and were maintained at ≥2 nmol/mg for 14 days, except for in T3, which showed a value of 1.71 nmol/mg. On all storage days, the carbonyl contents of T2 and T3 were significantly lower than that of the control (p<0.05). The carbonyl content of T3 reached a maximum at 8 days of storage and then decreased until 14 d of storage. A decreased carbonyl content was also observed in turkey muscle (Batifoulier et al., 2002) and beef homogenates (Mercier et al., 2004). The reduction of the carbonyl content is a further conversion of carbonyls into Schiff-base adducts (Li et al., 2019). Extracts from various plants such as pine bark and grape seed (Vuorela et al., 2005), white grape (Jongberg et al., 2011), and persimmon peel (Choe et al., 2017) showed similar inhibitory effects on pork patties, beef patties, and raw ground pork, respectively. Oxidative stress in muscle proteins induces oxidative degradation of some amino acid side chains (lysine, proline, arginine, and histidine) and increases the protein carbonyl content (Stadtman and Levine, 2003). Thus, the antioxidative effect of AHR decreased oxidative stress and inhibited carbonyl formation.
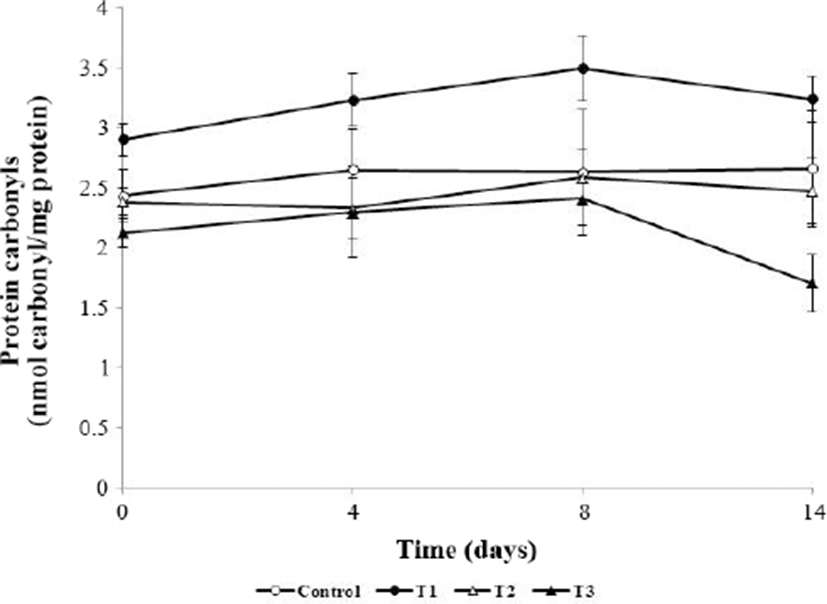
During the storage period, the sulfhydryl concentration decreased in all groups (Fig. 5). The initial sulfhydryl concentration in the control was higher than those in T2 and T3, but the differences were not significant (p>0.05). However, at 4 d, 8 d, and 14 d, T2 and T3 showed significantly lower sulfhydryl concentrations than the control (p<0.05). Protein oxidation induces protein crosslinking through formation of an intermolecular disulfide bridge, resulting in a decreased sulfhydryl concentration (Lara et al., 2011). These crosslinks induce protein aggregation, leading to decreases in the digestibility and water holding capacity (Batifoulier et al., 2002; Lund et al., 2011). However, the pro-oxidative effect of muscle foods during storage has been reported in several studies (Jongberg et al., 2011; Lara et al., 2011; Zhang et al., 2017). This phenomenon occurs because of the formation of covalent bonds of sulfhydryl groups and phenol following addition of phenolic compounds (Jongberg et al., 2013). However, the rate of disulfide bond formation during storage was low in the order of T3, T2, control, and T1 (4.9% < 26.6% < 31.2% < 72.6%, respectively). Therefore, the phenolic component of the AHR and sulfhydryl groups were combined to reduced sulfhydryl concentration. However, the rate of disulfide bond formation of the AHR-added meatball group was low, and analysis of the carbonyl content (Fig. 4) showed that addition of AHR to meatballs protected against protein oxidation during storage.
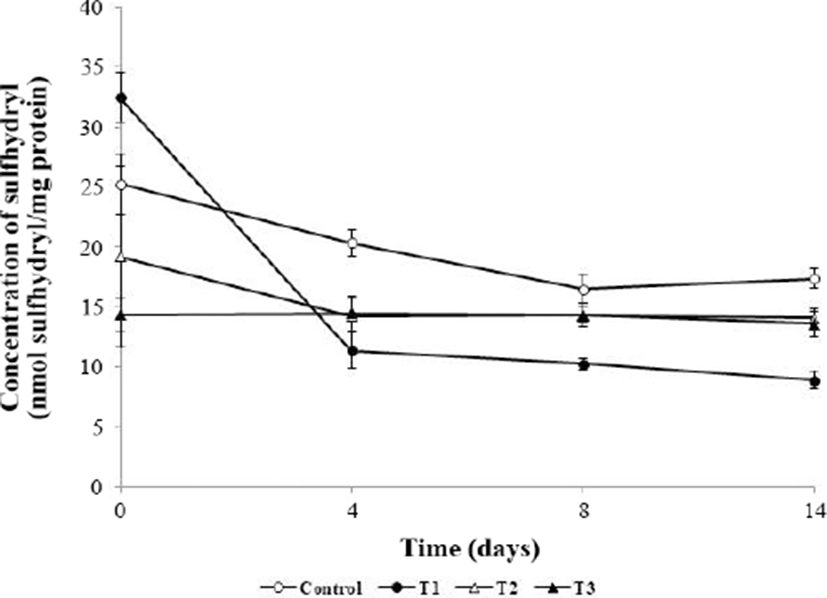
Conclusions
This study was conducted to investigate the effect of AHR on meatballs prepared from pork longissimus dorsi. The TBARS values, disulfide bond formation, carbonyl content, and VBN values of AHR-added meatballs were lower than those of the control; these results suggest that the antioxidant capacity of sulfur compounds in AHR can extend the storage period of meat products. Therefore, AHR can replace synthetic antioxidants in heated meat products. Further sensory evaluations by consumers or trained panelists are required to confirm the practicality of using AHR as an antioxidant in meat products.