Introduction
Coenzyme Q10 (CoQ10) is a vitamin-like compound present in all tissues of human body as both reduced form (ubiquinol-10) and oxidized from (ubiquinone-10). CoQ10 plays an essential role in the energy production of cells since it acts as an electron carrier in the lipid phase of mitochondrial membrane, which is necessary for ATP production (Bhagavan and Chopra, 2006). Moreover, CoQ10 exerts many health benefits due to its antioxidant activity and impacts on gene expression related to metabolism, and transport (Ercan and El, 2012; Barakat et al., 2013). Although CoQ10 is synthesized in human body, its synthesis is getting decreased with aging (Ercan and El, 2012). For example, the amount of CoQ10 in 70-year-old human is about 50% lower than that of 20-year-old human (Parikh et al., 2009). Therefore, the consumption of exogenous CoQ10 by food and supplements is important for elderly. However, the application of CoQ10 to food is often challenging because its low water solubility and high molecular weight (863 Da) resulting in poor bioavailability (Barakat et al., 2013). For the successful application in food system as well as improvement of oral bioavailability of CoQ10, it is crucial to manufacture an effective delivery system for CoQ10, which can improve its solubility, stability, and bioavailability.
For the oral consumption of CoQ10, lipid-based delivery systems, especially emulsions, have been studied to improve the bioavailability of hydrophobic bioactive compounds (Barakat et al., 2013; Lee et al., 2013). However, conventional emulsions could not often enhance the oral bioavailability of bioactive compounds possibly due to its relatively large droplet size (>1 μm) (Thanatuksorn et al., 2009). In human study, oil-in-water (O/W) emulsions with droplet size about 2.4 μm did not significantly increase the oral bioavailability of CoQ10 (Thanatuksorn et al., 2009). Compared to conventional emulsions, nanoemulsions have smaller droplet size with larger surface areas, which make them highly stable against coalescence and sedimentation during storage (Solans et al., 2005) and efficiently interact with biological component, such as intestinal pancreatic lipase (Carli et al., 2005; Salvia Trujillo et al., 2013).
Bioaccessibility (BA) refers as the proportion of compounds, which can be released and solubilized from a food matrix during digestion in gastrointestinal tract (Gomez-Estaca et al., 2019). Those soluble fractions become available for intestinal absorption (Carrillo et al., 2017). Since those soluble fractions could be absorbed in small intestine, the measurement of BA is important to predict the bioavailability of food formulations (Gomez-Estaca et al., 2019; Salvia-Trujillo et al., 2013). In vitro digestion experiments performed in this study can be easy and rapid approaches to measure the BA of bioactive compounds.
In our previous study, CoQ10 was successfully encapsulated in β-lactoglobulin (β-lg) nanoemulsions with a droplet size about 170 to 220 nm using an internal gelation method (Lee et al., 2013). During the formation of nanoemulsion, the intermolecular association of β-lg played an important role in determining droplet size. It was reported that the use of heat treatment and addition of CaCl2 may affect the intermolecular associations of β-lg at emulsion surface (Sok Line et al., 2005). The heat treatment of β-lg above 60℃ induces partial unfolding and increases the surface hydrophobicity of proteins, which can enhance interactions between β-lg molecules (Lee et al., 2013). On the other hand, the addition of CaCl2 as a cross-linking reagent can enhance the intermolecular associations of β-lg and promote gelation process at the oil and water interface in β-lg emulsions (Sok Line et al., 2005). Therefore, heat treatment and addition of CaCl2 affecting the intermolecular associations of β-lg may modulate the droplet size of β-lg nanoemulsions. In this study, it is hypothesized that the heat treatment of β-lg and use of CaCl2 as a cross-liking agent to modulate the droplet size of β-lg nanoemulsions can be a key factor to influence the BA of CoQ10.
The aims of this research were to investigate the effect of manufacturing variables, such as heating temperature and CaCl2 concentration level, on the physicochemical characteristics of β-lg nanoemulsions and to study how the droplet size of nanoemulsions affects the BA of CoQ10 during in vitro digestion.
Materials and Methods
β-lg was generously provided by Davisco International, Inc. (Le Sueur, MN, USA). CaCl2, polyoxyethylene (20) sorbitan monooleate (Tween 80), CoQ10, pocine gastric mucin, NaCl, lipase, and bile extract were obtained from Sigma-Aldirich Inc. (St. Louis, MO, USA).
β-lg nanoemulsions containing CoQ10 were produced by modified internal gelation method (Beaulieu et al., 2002; Lee et al, 2013). β-lg solutions (1%, w/v) were adjusted to pH 7.0 and pre-heated at 65, 75, or 85℃ for 10 min. To form O/W nanoemulsions, pre-heated β-lg solutions containing Tween 80 (5%, w/v) were mixed with grape seed oil containing CoQ10 (0.5 mg/mL) at an aqueous-oil ratio of 3:1. β-lg solution/oil mixtures were then homogenized at 6,000 rpm for 5 min using a homogenizer (Daihan scientific Co, Korea) followed by three power levels of sonications at 30, 50, and 70 W for 3 min using a probe-type ultrasonic sonicator (Bandelin Co, Germany). Finally, CaCl2 was added to 12 mL of β-lg solution/oil mixtures to a final CaCl2 concentration of 3, 4, or 5 mM.
Transmission electron microscopy (TEM, FEI Tecnai 12, Philips, Netherlands) was used to study the formation and morphological properties of β-lg nanoemulsions. Nanoemulsions were diluted 10-fold with deionized water. Twenty microliters of diluted nanoemulsions were placed onto a 200 mesh copper grid covered with carbon and stained with 2% uranly acetate solution for 15 s. TEM images of nanoemulsions were obtained at 120 kV.
The droplet size (Z-average), polydispersity index (PDI), and zeta-potential values of β-lg nanoemulsions were assessed using Zetasizer Nano ZS (Malvern Instruments, UK) with a scattering angle of 173° and a laser beam at 633 nm. Prior to analysis, nanoemulsions were 10-fold diluted with 1% (v/v) Tween 80 solution to disperse nanoemulsion droplets. The Smoluchowski equation was used to obtain zeta potential values from the electrophoretic mobility (velocity of a particle in an electric field, μm/s) of nanoemulsions.
To investigate in vitro digestion of β-lg nanoemulsions containing CoQ10, three-phase gastrointestinal tract model was used (Salvia-Trujillo et al., 2013; Zou et al., 2016). This model consists of mouth, stomach, and small intestinal phases to mimic human digestion processes. For digestion in mouth phase, 5 mL of β-lg nanoemulsion was mixed with 5 mL of simulated oral fluid (0.2% porcine mucin in 0.1 M NaCl, final pH: 6.8) and then incubated at 37℃ for 30 min with shaking at 100 rpm (water-bath, WiseCube® model WIS-20R, Daihan scientific Co, Korea). For stomach phase, 5 mL of mixture from mouth phase was added to 5 mL of simulated gastric fluid (0.2% NaCl, 0.32% pepsin, final pH: 2.5) followed by incubation at 37℃ for 2 h with shaking. Finally, 5 mL of mixture from stomach phase was added to 5 mL of simulated intestinal fluid containing bile extract and lipase (pH 7.0) followed by incubation at 37℃ for 2 h. The final concentrations of bile salts and lipase were 5.0 mg/mL and 1.6 mg/mL, respectively.
The BA of CoQ10 in β-lg nanoemulsions was evaluated by the modified method of Qian et al. (2012) and Salvia-Trujillo et al. (2013). Briefly, 10 mL of digested emulsions through three-phase digestion procedures was centrifuged at 10,000×g at 4℃ for 35 min. After centrifugation, transparent bioaccessible fractions of CoQ10 (clear micelle phase) were obtained at the middle. Digested emulsions (row digesta form) of CoQ10 before centrifugation and transparent fractions of CoQ10 (clear micelle phase) after centrifugation were collected. To analyze the amount of CoQ10 in raw digesta and micelle phase, collected fractions of row digesta and micelle phases were filtered with a 0.45 μm syringe filter and dissolved in chloroform. CoQ10 content in chloroform phase was assessed using quantitative spectrophotometric analysis (Terroso et al., 2009). Absorbance was determined at 280 nm and the amount of CoQ10 was calculated by a standard curve. The BA of CoQ10 was computed by following equation (Salvia-Trujillo et al., 2013).
All data were expressed as mean ± standard errors of three replicates. One-way analysis of variance (ANOVA) with the Fisher’s least significant difference (LSD) test was used to determine the effects of heating temperature and CaCl2 concentration level on the physicochemical properties including droplet size, polydispersity index, and zeta-potential value, and BA of β-lg nanoemulsions containing CoQ10. The significance level was set at the 5% level (p<0.05). Effects of manufacturing variables (heating temperature and CaCl2 concentration level) on the physicochemical characteristics of β-lg nanoemulsions during in vitro digestion were analyzed using two-way nested ANOVA. Pearson’s correlation coefficient analysis was performed to assess the relationships between the droplet size of β-lg nanoemulsions and BA of CoQ10. All statistical analyses were performed with SAS software package (2003).
Results and Discussion
β-lg nanoemulsions containing CoQ10 were manufactured by an internal gelation method with CaCl2. Fig. 1 exhibits the droplet size of β-lg nanoemulsions. As heating temperature was increased from 65 to 85℃, a significant (p<0.05) increase in the droplet size of nanoemulsions from 183 to 219 nm was observed (Fig. 1A). It was reported that β-lg undergoes partial unfolding upon heating above 65℃, which leads to the exposure of hydrophobic residues and free sulfhydryl groups buried inside of β-lg molecules (Lee et al., 2008; Lee et al., 2013). Exposed hydrophobic residues and free sulfhydryl groups of β-lg could then contribute to an increase in interdroplet interactions among β-lg adsorb on the surface of fat globules or nonadsorbed β-lg in continuous phase via hydrophobic attractions and disulfide bridging (Euston et al., 2000; Keowmaneechai and McClements, 2006; Raikos, 2010). When β-lg was treated at higher heating temperature, interactions between β-lg molecules could be increased. It may enhance the thickness and aggregation of fat globules, which can contribute to an increase in the droplet size of β-lg nanoemulsions at higher heating temperature.
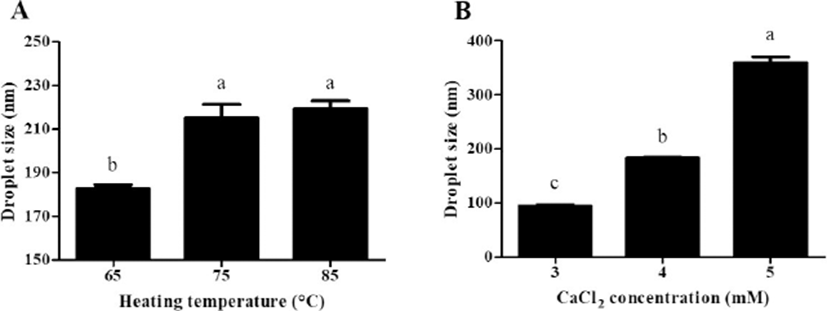
When CaCl2 concentration level was increased from 3 to 5 mM, a significant (p<0.05) increase in the droplet size of β-lg nanoemulsions from 94 to 360 nm was observed (Fig. 1B). At pH 7.0, there were electrostatic repulsions between β-lg molecules due to the negative surface charges of β-lg molecules (Harnsilawat et al., 2006). The addition of CaCl2 to β-lg-solutions can reduce electrostatic repulsions between the negative charged groups of β-lg molecules, which could enhance associations between β-lg molecules (Barbut and Foegeding, 1993; Chen and Subirade, 2006; George and Abraham, 2006). These Ca2+-induced associations may lead to an increase in interfacial adsorption and interactions among adsorbed β-lg molecules and fat globules, which resulted in an increase in the droplet size of nanoemulsions at higher CaCl2 concentration.
There were no significant (p<0.05) differences observed in the polydispersity index (Figs 2B and 3B) and zeta-potential values (Fig. 5) of initial β-lg nanoemulsions manufactured with various heating temperatures and CaCl2 concentrations. The PDI values of initial β-lg nanoemulsions were below 0.3, which indicates that initial nanoemulsions had homogeneous droplets (Dragicevic-Curic et al., 2010; Yen et al., 2010). The zeta-potential values of initial β-lg nanoemulsions rainging from –7.9 to –8.9 mM also imply that β-lg nanoemulsions had negative surface charges.
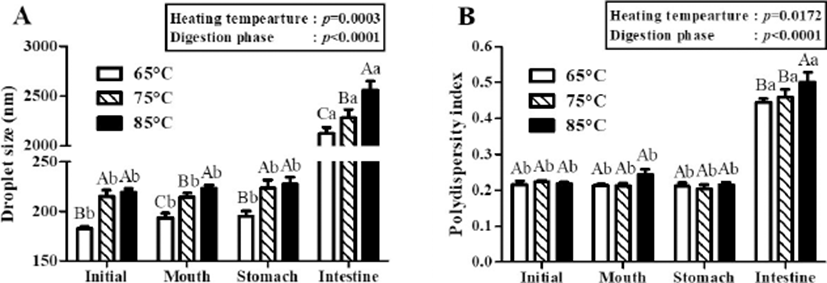
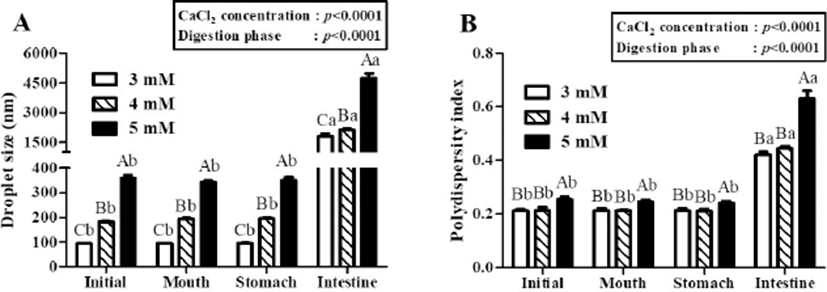
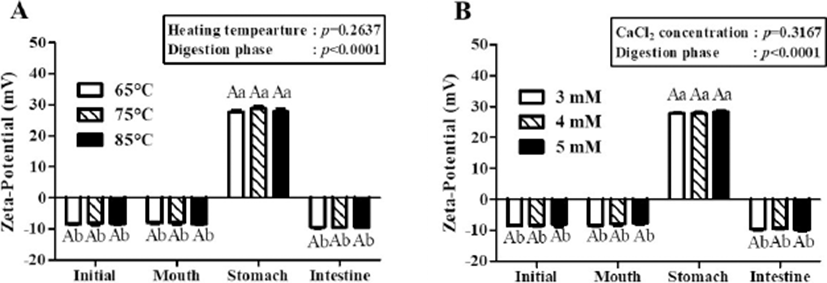
The physicochemical characteristics of β-lg nanoemulsions during three-stage in vitro digestions were monitored. In this study, β-lg nanoemulsions were produced with grape seed oil, which contained mainly triglycerides and hence was digestible in gastrointestinal tract (Baydar, 2001). Fig. 2 presents the changes in the droplet size and PDI value of β-lg nanoemulsions treated with various heating temperatures during in vitro digestion. Two-way nested ANOVA results revealed that heating temperature (p=0.0003) and digestion phase (p<0.0001) had significant factors to affect the droplet size of β-lg nanoemulsions during three-stage in vitro digestions (Fig. 2A) while the PDI value of β-lg nanoemulsions was also significantly affected by heating temperature (p=0.172) and digestion phase (p<0.0001) (Fig. 2B). After digestions in mouth (pH 6.8, 30 min) and stomach (pH 2.5, 2 h) phases, there were no significant (p<0.05) changes between the droplet size of β-lg nanoemulsions manufactured with 65, 75, and 85℃ while β-lg nanoemulsions were remained with PDI value below 0.3. It indicates that those β-lg nanoemulsions are stable in mouth and stomach phase (Fig. 2). Since β-lg is resistant to pepsin, nanoemulsions prepared with β-lg can be stable in stomach phase (Chen and Subirade, 2005; Kitabatake and Kinekawa, 1998). After digestion in intestinal phase (pH 7.0, 2h), a significant increase (p<0.05) in the droplet size (>2,000 nm) of β-lg nanoemulsions was observed (Fig. 2A) implying that those nanoemulsions were unstable to droplet coalescence and aggregation in intestinal phase. Since β-lg can be hydrolyzed in the intestine (Kitabatake and Kinekawa, 1998), the physical stability of nanoemulsions was reduced. The PDI values of β-lg nanoemulsions in intestinal phase were significantly (p<0.05) higher than PDI values in mouth and stomach phases (Fig. 2B) indicating that nanoemulsions were changed from monodispersed (homogeneous) state in initial, mouth, and stomach phase to polydispersed (inhomogeneous) state in intestinal phase. These results on droplet size and PDI values suggested that there were significant changes in the physical stability of nanoemulsions. Digestion of nanoemulsions may alter the interfacial and core properties of lipid droplets, which may enhance droplet aggregation (flocculation or coalescence) (Ozturk et al., 2015).
In two-way nested ANOVA, CaCl2 concentration level (p<0.001) and digestion phase (p<0.001) were key factors to affect the droplet size and PDI values of β-lg nanoemulsions during three-stage in vitro digestion (Fig. 3). There were no significant (p<0.05) changes between the droplet size and PDI values of β-lg nanoemulsions treated with 3, 4, and 5 mM CaCl2 concentration level after digestions in mouth and stomach phases while a significant (p<0.05) increase in the droplet size and PDI values of β-lg nanoemulsions were observed in intestinal digestion phase (Fig. 3). These results imply that β-lg nanoemulsions prepared with various CaCl2 concentration levels had good stabilities in mouth and stomach phases, whereas poor stability was observed in intestinal phase due to lipid digestion.
In this study, it seems that initial droplet size affected the droplet size of β-lg nanoemulsions in intestinal phase. For example, β-lg nanoemulsions prepared with 3 mM and 5 mM CaCl2 had initial droplet size of 93 and 348 nm, respectively (Fig. 3A). The droplet size of these nanoemulsions was significantly (p<0.05) increased in intestinal phase (about 1,800 nm for 3 mM CaCl2 and about 4,700 nm for 5 mM CaCl2, respectively). β-lg nanoemulsions with larger initial droplet size exhibited larger droplet size and were more polydisperse (inhomogeneous) in intestinal phase indicating that they had lower stability to aggregation. Those changes were also supported by TEM micrographs (Fig. 4). β-lg nanoemulsions in mouth and stomach phases had similar morphology while large changes in the morphology and droplet size were observed in simulated intestinal phase (Fig. 4). Since nanoemulsions with smaller droplet size may provide more surface areas for lipase, a reduction in the initial droplet size and PDI value of nanoemulsions could be a very useful way to make them more favorable to be digested in small intestine by lipase. It may enhance the release and solubilization of entrapped hydrophobic bioactive compounds. Similar results were observed by Salvia-Trujillo et al. (2013), which reported that O/W Tween 20 emulsions with smaller initial droplet size (~200 nm) had smaller droplet size in simulated intestinal fluid compared with emulsions with larger initial droplet (~23,000 nm).
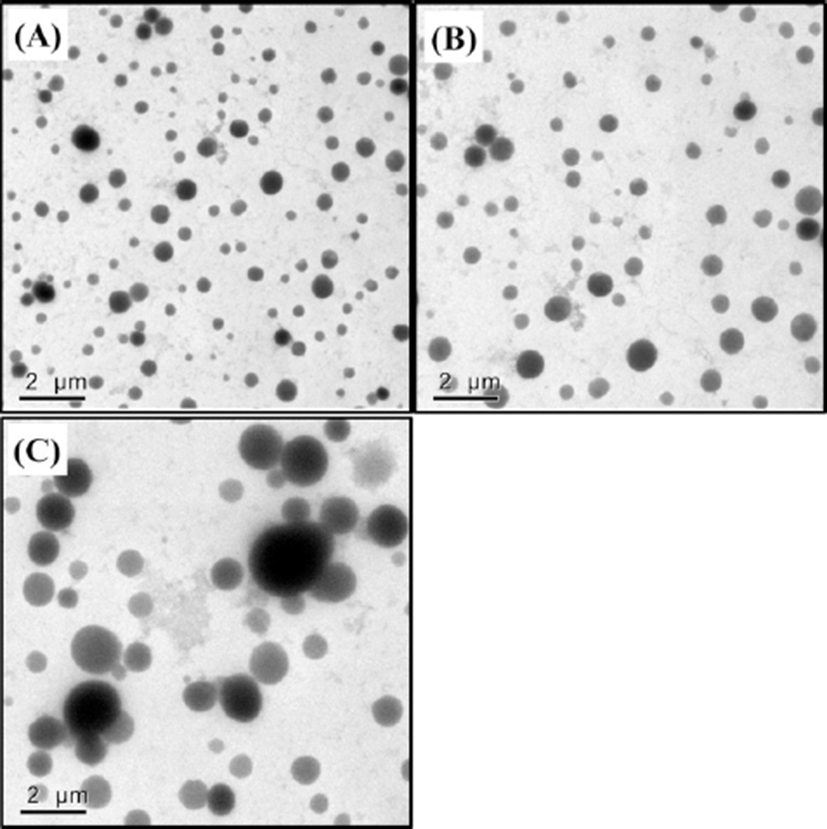
The zeta-potential values of β-lg nanoemulsions during three-stage in vitro digestion were presented in Fig. 5. In two-way nested ANOVA results, no significant impacts of heating temperature and CaCl2 concentration level on the zeta-potential value of β-lg nanoemulsions were observed while digestion phase significantly (p<0.001) affected the zeta-potential value of β-lg nanoemulsions. β-lg nanoemulsions treated with various heating temperatures (Fig. 5A) and CaCl2 concentration levels (Fig. 5B) had zeta-potential values about -8 mV during digestion in mouth phase. However, the zeta-potential values of those β-lg nanoemulsions were significantly (p<0.005) enhanced in stomach phase (pH 2.5) indicating that the surface charges of β-lg nanoemulsions were shifted from negative to positive during digestion in stomach phase. Since there was a change in pH from 6.8 (mouth phase) to 2.5 (stomach phase) and isoelectric pH β-lg is around pH 5.2 (Chen and Subirade, 2005), β-lg nanoemulsions had surface charge shift from negative to positive. During digestion in intestine phase (pH 7.0), the surface charges of β-lg nanoemulsions were changed from positive to negative with zeta-potential values about –9.6 mV.
Impacts of heating temperatures and CaCl2 concentration levels on the BA of CoQ10 after in vitro digestion were presented in Fig. 6. As heating temperature was increased from 65℃ to 85℃, the BA of CoQ10 was significantly (p<0.05) decreased from 50% to 41% (Fig. 6A). The BA of CoQ10 was evaluated by measuring CoQ10 content in the fraction of water-soluble mixed micelles after three-stage in vitro digestion (Qian et al., 2012). Before in vitro digestion, CoQ10 was solubilized in oil droplets of β-lg nanoemulsions, which could be hydrolyzed into free fatty acids by pancreatic lipase. Free fatty acids and bile salts form mixed micelles with CoQ10 and those micellar CoQ10 could be absorbed by intestinal epithelial cells (Salvia-Trujillo et al., 2013; Wang et al., 2012). As shown in Fig. 1A, the initial oil droplet size of β-lg nanoemulsions was decreased from 219 to 183 nm with decreasing heating temperature from 65℃ to 85℃. A reduction in the initial size of oil droplet may promote lipid digestion and micelle formation in intestinal phase since smaller droplet size had larger surface areas available for pancreatic lipase action. An increase in the surface areas of oil droplets could offer more surfaces that the lipase can act and hence could promote the formation of mixed micelle of hydrophobic bioactive compounds, such as β-carotene and CoQ10 (Qian et al., 2012; Wang et al., 2012). Therefore, β-lg nanoemulsions treated at lower heating temperature (i.e., 65℃) with smaller initial droplet size had higher BA of CoQ10. Similar results were observed for β-lg nanoemulsions prepared with various CaCl2 concentration levels. In Figs 1B and 6B, β-lg nanoemulsions treated with 3 mM CaCl2 exhibited the initial droplet size of 94 nm and BA of CoQ10 about 57% while β-lg nanoemulsions prepared with 5 mM CaCl2 had the initial droplet size of 360 nm and 39% of CoQ10 BA. There were significantly (p<0.01) negative correlations between the initial droplet size of β-lg nanoemulsions and BA of CoQ10 with r values of –0.76 (Fig. 7). This result suggests that the initial droplet size of β-lg nanoemulsions can be a key factor to affect the BA of CoQ10. Similar results were reported for carotenoid in O/W emulsions prepared with Tween 80. A reduction in the size of emulsion resulted in an increase in the BA of carotenoids (Salvia-Trujillo et al., 2017).
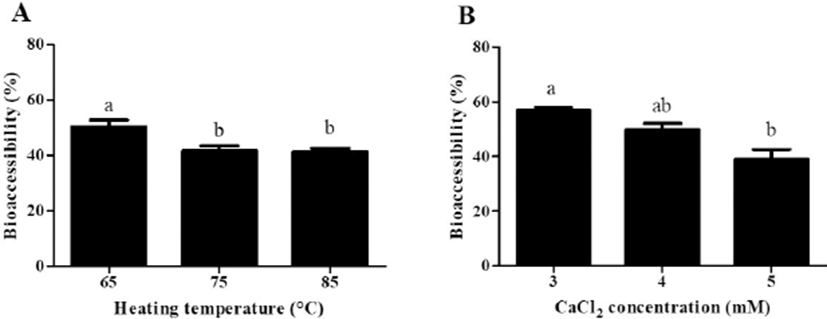
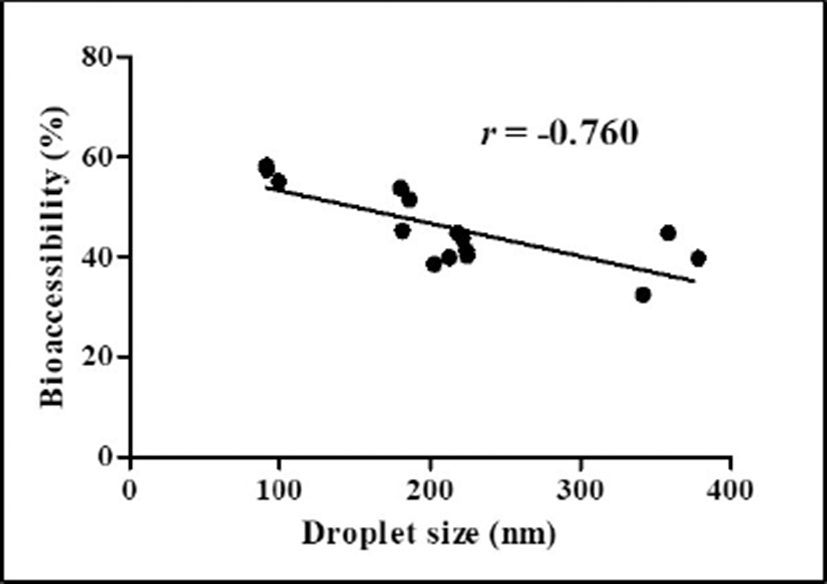
Conclusions
In conclusions, β-lg nanoemulsions containing CoQ10 were successfully manufactured using the modified internal gelation method. Heating temperature and CaCl2 concentration level were key manufacturing variables to determine the initial droplet size of β-lg nanoemulsions and BA of CoQ10. During in vitro digestion, initial droplet size was not changed in simulated mouth and stomach phases while a meaningful increase in droplet size was observed in simulated intestinal phase. Negative correlations between the initial droplet size of β-lg nanoemulsions and BA of CoQ10 were observed indicating that the initial droplet size of β-lg nanoemulsions could be a crucial aspect to determine the BA of CoQ10. This study will contribute to better understanding of factors affecting the BA of entrapped bioactive compounds in nanoemulsions and help to allow food manufacturer to use β-lg nanoemulsions as a delivery system in functional foods.