Introduction
White meat, such as chicken and pork, are considered superior to red meat in terms of human health, due to their comparably lower fat and higher protein contents. Consumer perceptions, as well as the associated lower prices, convenience, and lack of religious restrictions further aid in their popularity and consumption (Jaturasitha et al., 2008). Nutritional value and meat quality are influenced by several factors; such as age, body weight, growth performance, and environmental conditions (Jung et al., 2011). Chicken meat also contains numerous endogenous bioactive compounds, which, in routine dietary inclusion, can reduce the incidence of many diseases and provide several health benefits (Jayasena et al., 2013).
The bioactive compound; such as choline, betaine, L-carnitine, creatine, and taurine are essential nutrients related to brain development, the metabolism of fatty acids, osmoregulatory properties, supply energy to muscles, and for the regulation of the central nervous system (De Zwart et al., 2003; Flanagan et al., 2010; Li et al., 2015; Mora et al., 2010; Wu and Shiau, 2002; Wyss and Kaddurah-Daouk, 2000) and have been shown to possess health-promoting characteristics. Free amino acids (FAAs) contribute to the taste of many foods (Zhao et al., 2016). Additionally, glutamic acid, a savory amino acid, is well known for its umami taste, and is one of the most important amino acids in chicken meat (Ali et al., 2019).
Several studies have shown that Korean indigenous chickens possess higher amounts of bioactive compounds than commercial broiler chickens, which are affected by genotype, muscle fiber, meat portion, gender, age, and cooking method (Ahn and Park, 2002; Jayasena et al., 2014; Jayasena et al., 2015; Jung et al., 2013). Similarly, the meat from Japanese native chickens is considered more palatable than that of typical broiler chickens, due to its high nutritional value (Rikimaru and Takahashi, 2010). In Thailand, four main commercial chicken are raised: broiler; spent hen; black-boned chicken; and the Thai native chicken. Broilers, which are generally imported, have high growth performance and a low cost of production and have become a staple meat-product in Thai markets. Spent hen, another potential source of chicken meat, is discharged at the age of 80 to 100 wk. The texture of spent hen is tough due to the accumulation of collagen (Chuaynukool et al., 2007); however, spent hen chickens are occasionally fattened in Thailand to complement indigenous chickens in times of high demand for meat of indigenous origin (Jaturasitha et al., 2008). The black-boned chicken and Thai native chicken, which are indigenous strains, have slow growth rates, which limit their production. While some Thai consumers prefer the taste of meat from native chickens; the market is small, although gaining in popularity (Wattanachant et al., 2005). Raised in rural and mountainous areas of Thailand, black-bone chickens have other special properties and their skin, bones, and meat are black (Tu et al., 2009), which accounts for their notable consumer demand (Jaturasitha et al., 2008).
Numerous studies of chicken meat in Thailand have focused on growth performance, carcass quality, and meat quality; including our former study on the macronutrient composition and antioxidant capacities of chicken breast meat. While the findings showed that the chemical composition, amounts of protein, and the antioxidant biomarkers, including carnosine and anserine, were affected by genotype; little information has been provided on the nutritional properties and bioactive compounds of different chicken breeds. The objective of the present study was to compare the unique taste, nutritional properties and endogenous bioactive compounds across four chicken genotypes: commercial broiler; spent hen; black-boned; and Thai native. We intend that the results may be used to identify which genotypes would be most suitable to certain situations, resulting in the promotion of meat production, as well as influencing consumer consumption.
Materials and Methods
Each of the four genotypes of chicken in this study; broiler, spent hen, Thai native, and black-boned, were reared in one flock on a single farm under identical conditions. The chickens were fed formulated diets obtained from a commercial feed, according to their genetic requirements. Feed and water were provided for ad libitum intake. The broilers were fed until they were six weeks old; whereas spent hen, black-boned chicken, and Thai native chicken were fed until they were 72 wk old, 20 wk old, and 16 wk old; respectively. The average live weights of each chicken genotype were 2.1±0.2 kg (broiler), 1.7±0.1 kg (spent hen), 1.2±0.1 kg (black-boned), and 1.3±0.1 kg (Thai native). At the end of the experiment, ten chickens of each genotype were slaughtered using standard methods. Their carcasses were chilled at 4°C for 24 h, and the breast meat without fat was immediately separated. The breast meat was then minced with a meat grinder, immediately cooled in an ice bath, and stored at –20°C before freeze-drying. Freeze-dried meat samples were ground to powder using a mortar and pestle and stored at –20°C before analyses.
The FAA and taurine contents were measured according to the method of Bidlingmeyer et al. (1984) with some modifications. Each sample was mixed with 10 mM hydrochloric acid (HCl) and acetonitrile (ACN). The homogenate was centrifuged at 10,000×g for 10 min at 4°C (TOMY MX-301, Tokyo, Japan). The supernatant was neutralized by adding a mixture of methanol (MeOH)/water/triethylamine (TEA) (2:2:1, v/v); then dried completely in a vacuum. Then, the samples were dissolved with a mixture of MeOH/water/TEA/phenyl isothiocyanate (PITC) (7:1:1:1, v/v) for derivatization and incubated for 20 min for phenylthiocarbamyl amino acid production. The mixtures were then filtered through a 0.45 μm PVDF syringe filter (Whatman International, Maidstone, UK). Phenylthiocarbamyl amino acids and taurine were separated using an HPLC system with an L-column3 C18, 5 μm particle size (250×4 mm; Nacalai Tesque, Kyoto, Japan). A binary linear gradient was used with 100% ACN as mobile Phase A, and 150 mM ammonium acetate, pH 6.2 containing 5% ACN as mobile Phase B at a flow rate of 0.6 mL/min. The column temperature was maintained at 40°C. The gradient program was as follows: 0–3 min, 0%–6% B; 3–20 min, 6%–22% B; 20–25 min, 22%–60% B; 26–37.1 min, 100%–0% B; 37.1–50 min, 0% B. The separation was monitored using a diode array detector at wavelengths of 214 and 254 nm.
The total amino acid contents of the samples were determined by the method outlined by Bidlingmeyer et al. (1984) with slight modifications. The samples were digested with 6 N HCl at 150°C for 1 h. The resultant amino acids were derivatized with PITC, and the PTC-amino acids were resolved using the same method as that for the FAAs.
The betaine, carnitine, creatine, and choline contents were determined through liquid chromatography-electrospray ionization-tandem mass spectrometry (LC-MS/MS, LCMS 8040; Shimadzu, Kyoto, Japan). The standards and samples were dissolved in distilled water and centrifuged at 10,000×g for 20 min. The supernatant was then mixed with ethanol and filtered. An Inertsil ODS-3 column (2 mm inner diameter×250 mm; GL Science, Tokyo, Japan) was used for the LC separation. The column temperature was controlled at 40°C. The mobile Phase A contained 0.1% formic acid, and B contained 0.1% formic acid in 80% ACN. The gradient program used was as follows: 0–10.01 min, 100% B; 10.01–15.01 min, 100% B; and 15.01–25 min, 0% B. The flow rate of the mobile phase was 0.2 mL/min. The total ion intensity was monitored in a positive mode. Several scan modes, including a precursor ion scan, product ion scan, and multiple reaction monitoring were used to quantify the betaine L-carnitine, creatine, and choline contents.
All experiments were carried out as mean±SD of three independent measurements and were subjected to One-way Analysis of Variance (ANOVA), and the significance of mean differences was determined by the Duncan Multiple Range test using SPSS version 23.0 (SPSS Inc., Chicago, IL, USA), in which p-value less than 0.05 were considered significant.
Results and Discussion
FAAs have long been associated with the characteristic tastes of food (Wu and Shiau, 2002). The FAA concentrations of breast meat in broiler, spent hen, black-boned, and Thai native chickens are presented in Table 1. For essential amino acids, the dominant FAAs of all genotypes were leucine and valine, whereas threonine was found in higher amounts in broiler and black-boned chickens (p<0.05). Alanine, a non-essential amino acid, was greatest in all genotype contents (p<0.05). Alanine was the predominant non-essential amino acid in chicken fillets (Ali et al., 2019). Furthermore, asparagine showed the lowest content in broilers, whereas the highest content was found in the spent hen, Thai native, and black-boned chickens (p<0.05). Glutamic acid is one of the most important amino acids in chickens, which enhances the palatability of chicken meat (Rikimaru and Takahashi, 2010). In the present study, glutamic acid content was the highest in Thai native chickens, followed by black-boned, broiler, and spent hen chickens (p<0.05). These results agree with the study of Wattanachant et al. (2004) which indicated that the glutamic acid contents in native chickens were higher than those in broilers. Asparagine, threonine, serine, glutamic acid, glycine, and alanine have been classified as tasty amino acids (Ali et al., 2019), which were most prevalent in Thai native, broiler, black-boned, and spent hen chickens, respectively (Fig. 1). Flavor-related amino acids (valine, isoleucine, leucine, phenylalanine, arginine, proline, and methionine) are related to the tangy flavor in meat (Ali et al., 2019; Meinert et al., 2009). These flavor-related amino acids were found to be highest in content in black-boned chicken, and lowest in Thai natives and broilers (Fig. 1). Arginine, which is associated with an undesirable flavor complexity (Schiffman and Dackis, 1975), was highest in the black-boned chicken. This suggests that different genetic variants of chickens may be associated with different free-amino acids. According to Mir et al. (2017) indigenous chickens are higher in flavor and taste compounds than broilers, due to variations in their content of amino acids; including aspartic acid, threonine, serine, glycine, alanine, tyrosine, lysine, and arginine.
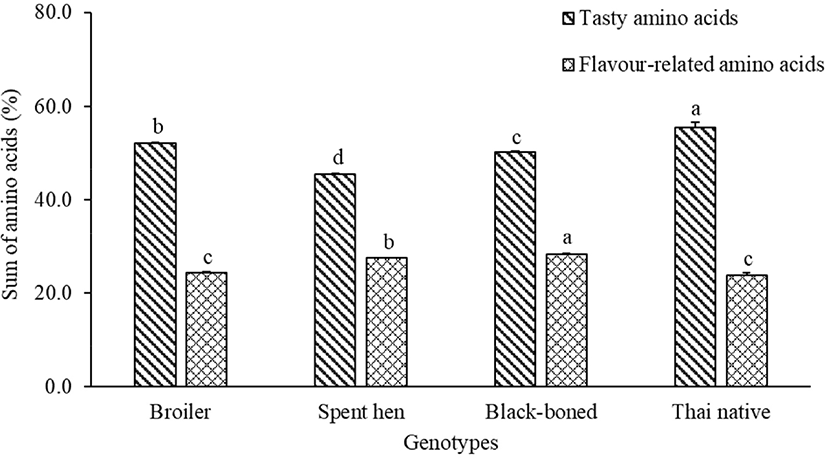
The amount of choline was significantly different in spent hen, black-boned, and Thai native chickens, compared to that of the broiler (p<0.05). Black-boned chicken also had the highest choline content, while spent hen and Thai native chickens had the lowest (Fig. 2A). Any variability found among the samples could be attributed to the differences of genetic origin. Broilers had 2.7-fold and 8-fold higher choline contents than spent hen and Thai native chickens, respectively. Cohen et al. (1995) suggested that the uptake of circulatory choline decreased with age. Within the present study, all chickens were slaughtered at market age, in which the spent hen and Thai native chickens were older than the broilers. Therefore, the different choline contents in our results may be due to slaughter age. The effect of chicken genotype on the amount of betaine was significantly lower across all genotypes in comparison to the broilers (p<0.05), shown in Fig. 2B. Jayasena et al. (2015) also determined that broilers had significantly higher betaine contents in Korean native chickens, which also decreased with chicken age (Jayasena et al., 2014). Our results confirmed these findings, in which spent hen chickens had the lowest betaine content compared to other genotypes (p<0.05). Moreover, betaine is synthesized by the oxidation of choline in mitochondria through betaine aldehyde dehydrogenase (Meier and Seitz, 2008). It could be assumed that high choline content leads to an increase in betaine content. Thus, the black-boned chicken showed significantly higher betaine content than the Thai native chicken (p<0.05). The amount of L-carnitine content was significantly different in spent hen, black-boned, and Thai native chickens, compared to that of the broiler chickens (p<0.05), but it did not significantly differ between black-boned and Thai native chickens (Fig. 2C). The L-carnitine content was different in chicken meat due to myofiber type (Shimada et al., 2004) regardless of the age of the chickens (Jayasena et al., 2014). However, it has been reported that the Thai native chicken had significantly higher muscle fiber Types I and IIA than imported fast-growing breeds, such as the Rhode Island Red chicken (Jaturasitha et al., 2008). Type I and IIA fibers contained greater amounts of mitochondria and produced higher levels of acetyl groups than Type IIB fibers. Thus, they need higher L-carnitine content to buffer the excess acetyl groups. This may explain why Thai native and black-boned chickens have high L-carnitine contents. Moreover, the impact of over-supplied L-carnitine may depend on its endogenic biosynthesis from lysine and methionine, which are essential amino acids (Ghoreyshi et al., 2019). Our findings showed that lysine and methionine contents of spent hens were lower than those of the broiler, black-boned, and Thai native chickens (Table 2). We may, therefore, conclude that the lower amounts of these amino acids in spent hens result in lower L-carnitine contents. Creatine and its derivative creatine phosphate play a pivotal role in muscle energy metabolism by donating its phosphate groups to adenosine diphosphate to regenerate adenosine triphosphate (Balsom et al., 1994; Wyss and Kaddurah-Daouk, 2000). The creatine content in chicken breast meat was not significantly different between genotypes (Fig. 2D). Several previous studies reported that numerous factors; such as age, cooking method, meat portion, and body weight, had no significant effect on the creatine content (Jayasena et al., 2014; Jung et al., 2013). Biosynthesis of creatine occurs in the liver, and is then distributed to skeletal muscles through creatine kinase activity, which catalyses the generation of phosphorylcreatine from creatine (Wyss and Kaddurah-Daouk, 2000). The taurine contents in all genotypes were significantly different from that in the broilers (Table 2). Black-boned chicken contained the highest amount of taurine, followed by broiler and Thai native chicken (p<0.05). Notably, the spent hen in our study, which was the oldest at 72 wk, produced no taurine content in their breast meat (p<0.05). The major route for the biosynthesis of taurine is from methionine and cysteine through cysteine sulfinic acid decarboxylase (Scicchitano and Sica, 2018). Wu and Shiau (2002) reported that high amounts of taurine can also be found in dark meat, which coincides with our findings of high taurine content in black-boned chickens. Similarly, the Thai native chicken showed low taurine contents compared to the broiler, due to their higher lightness (Wattanachant et al., 2004).
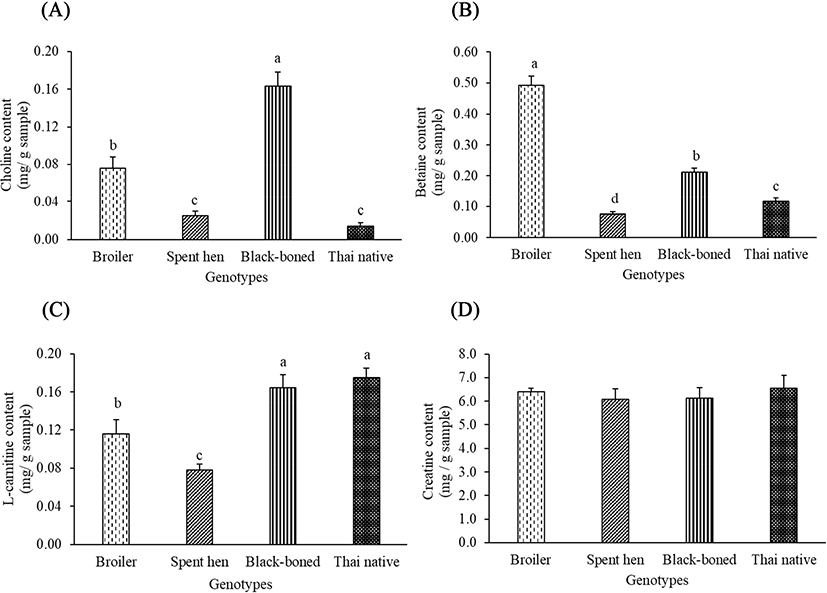
The amino acid profile is considered the most crucial nutritional property concerning consumer perceptions of meat. The total amino acid compositions of breast meat from four genotypes of chicken are shown in Table 2, in which seventeen amino acids were detected in this experiment, which differed significantly (p<0.05), except for alanine and tyrosine. Moreover, leucine, lysine, and threonine were the major constituent amino acids within all genotypes. We further noted that leucine content was significantly higher in black-boned and Thai native chickens than in broilers (p<0.05). The lysine content of the Thai native chicken was also significantly higher than in the broilers. The Thai native chicken also contained the highest threonine and glutamic acid contents among all genotypes (p<0.05), whereas cysteine content was lowest in the non-essential amino acid group. Thai native chicken had the highest glutamic acid content, followed by black-boned chicken, spent hen, and broilers (p<0.05). Meat is a source of food that has enriched branched-chain amino acids (Górska-Warsewicz et al., 2018). The study of Kim et al. (2017) found that arginine, leucine, and lysine were major essential amino acids in chicken meat, and were found in higher contents than other essential amino acids. We confirmed this in our findings, in that Thai native and black-boned chickens showed a higher content of essential amino acids compared with those of the commercial broiler. Several factors affected protein digestibility and deposition; such as species, age, and gender (Wu et al., 2014). The age and genotype of the chicken may be major factors that influence muscle composition. The Thai native chicken and black-boned chicken had slower growth rates than that of the broiler, and were classified as a ‘slow-growing’ type. The age difference may have affected protein deposition, due to the protein turnover rate (Tesseraud et al., 2000). Furthermore, the amount of lysine significantly differed between native chicken (120 d old) and broiler (42 d old) (Zhao et al., 2011). Therefore, our results indicate that levels of amino acid composition in breast meat are influenced by chicken genotype and slaughter age.
Conclusion
Our results showed that the levels of amino acids that were associated with the flavor and taste of chicken meat. The meat of the Thai native chicken and black-boned chicken were shown to be high in nutritional value and had some unique features and advantages over commercial broiler and spent hen chickens. We intend that the data from this study will provide valuable information concerning the functional meat for both Thai producers and consumers, where black-boned chicken proved to be an excellent source of nutrition, and Thai native chicken is both flavourful and luscious.