Introduction
Insect larvae have been investigated worldwide as future food resources; approximately 2,000 types of insects have been eaten globally. It is well known that insects and their larvae are high-quality protein-containing foods with favorable essential amino acid compositions and that they may replace meat as a dietary option (Belluco et al., 2013; Nowak et al., 2016). In Korea, larvae including Anacridium aegyptium, Gryllus bimaculatus, Bombyx mori, Protaetia brevitarsis seulensis, Tenebrio molitor and Trypoxylus dichotomus are officially approved by the Ministry of Food and Drug Safety, and registered as food resources.
Of particular interest, P. brevitarsis larvae have been traditionally applied in Korea as an effective agent for the treatment of adult diseases such as menstruation irregularities, stomatitis, tetanus, vision loss, cataracts, knife wounds, postpartum stroke, malignant boiling and cerebral stroke (Kang et al., 2000; Lee et al., 2017a). This larva has also been widely used as a traditional Chinese medicine and folk remedy because of excellent effects on liver diseases including liver cirrhosis, hepatitis, liver cancer and elimination of cumulative fatigue (Kang et al., 2000; Lee et al., 2017a). Recently, the safety of P. brevitarsis has been investigated in animal clinical trials using rats (Hwang et al., 2001; Yoon et al., 2007). In addition, changes in the chemical composition of these larvae (eg, nutrients, hazardous substances) have been evaluated (Chung et al., 2013; Noh et al., 2015), and beverages containing P. brevitarsis larvae extract have been tested for effectiveness and safety (Park et al., 2012).
P. brevitarsis have been successfully reared in an artificial breeding environment (Park et al., 1994) and their morphology and growth characteristics have been examined (Kang et al., 2005; Kim, 2005; Kim and Kang, 2005; Kim et al., 2005). In addition, feeds that are important during the rearing process have been studied with a fermented aloe replacement resource (Kang, 2011) and various supplementary feeds (Yoon et al., 2016), however, these studies have not comprehensively evaluated nutritional content.
This study was conducted to establish a rearing approach and evaluate the nutritional appropriateness of P. brevitarsis larvae as a functional animal resource by assessing the potential influence of various supplementary feeds on the chemical composition and abundance of bioactive materials.
Materials and Methods
P. brevitarsis larvae and oak-fermented sawdust (primary food source) were supplied from an agricultural corporation in Jinju, Gyeongnam, Korea. The larvae were reared in a rearing room (16L:8D, 25±2°C, 60±5% RH) at the insect farm of Gyeongnam National University of Technology and Science. Ten pairs of male and female were placed into a transparent polypropylene rectangular container (268×193×127 mm, 285×220×200 mm and 400×320×180 mm) and the larvae hatched from the oviposit eggs were employed for this study.
In order to investigate various supplementary feeds, aloe (Saponaria), apple (Busa), banana (Cavendish), sweet persimmon (Buyoo) and sweet pumpkin (Danhobak) were purchased from a traditional market in Korea. Supplementary feeds were sliced into 3 mm sections and positioned on top of fermented sawdust once a week (10, 15, or 20 g depending on the larval growth stage; Yoon et al., 2016). Remaining supplementary feed was removed 48 h after feeding. Since the 1st instar larvae were not change before and after the feeding assay, it was noted that supplementary food was not eaten. Therefore, the 1st instar larvae were excluded from the feeding assays. Supplementary feeds were supplied from the 2nd instar larvae, and the 3rd instar larvae in the second half were excreted in the fasted state for 3 days. Fasted larvae were washed twice with tap water, dried for 48 h at 60°C in a dryer, crushed with a grinder (Super grinder JL-1000, Hibell, Hwaseong, Korea), sorted with 0.5 mm sieve, kept in a freezer and then analyzed.
Chemical composition analysis of the crushed larval powder was conducted following fine pulverization at 4,600×g using a pin mill (DK201, Sejung Tech, Sejong, Korea). Chemical compositions were analyzed as described by the association of official agricultural chemists (AOAC, 2016). Crude fat and ash content were analyzed using the Soxhlet and direct-burn (550°C) methods, respectively. Crude protein content was quantitatively analyzed using a Kjeldahl analyzer (2300 Kjeldahl Analyzer Unit, Foss Tecator, Eden Prairie, MN, USA). Inorganic components were quantified using a dry method (Lee and Kye, 1970). Briefly, 1 g of sample was burn at 550°C and 0.5 N HNO3 was added. The solution was then: i) filtered [GF/C filter (90 mm, Cat No. 1822 090, Whatman International, Maiidstone, UK)], ii) adjusted into 50 mL with 0.5 N HNO3, and iii) analyzed by Inductively Coupled Plasma Spectrometer (ICP, Thermo Jarrell Ash, Franklin, MA, USA).
For analysis of fatty acid compositions, 0.5 g of the extracted crude fat was mixed with 2 mL of a reaction reagent (methanol:heptane:benzene:2,2-dimethoxypropane:H2SO4 =37:3:620:5:2 (v/v)), and then the mixture was heated to 80°C for 20 min. The separated supernatant was concentrated with nitrogen gas and dissolved in hexane; the resulting solution was used for analysis (Dewanto et al., 2002). Analysis was conducted using a gas chromatographer (Agilent 6850 GC, Agilent Technologies, Wilmington, NC, USA). An HP-INNOWAX column (30 m×0.25 mm, 0.25 μm, Aglient Technologies) and flame ionization detector were used; injector and detector temperatures were 250°C and 300°C, respectively. The oven temperature was maintained at 120°C for 5 min, raised to 230°C at increments of 5°C/min and held for 5 min at final temperature. N2 (99.999%) was used as the carrier gas, the flow rate was 1.3 mL/min and the final injection volume was 1 μL. Fatty acid composition was determined using the relative ratio of peak areas.
To analyze constituent amino acid compositions, an aliquot (1 g) of the dried P. brevitarsis larvae powder was mixed with 40 mL of 6 N HCl in a round flask, and then the mixture was hydrolyzed with nitrogen gas for 24 h at 110°C. After the hydrolyzed product was concentrated under a reduced pressure at 50°C, the concentrated solution was diluted with 50 mL of 0.2 N sodium citrate buffer (pH 2.2), and then filtered (0.25 μm Millipore filter). An aliquot (20 μL) of the filtered sample was analyzed using an amino acid analyzer (L-8900, Hitachi, Tokyo, Japan).
To analyze free amino acid content, an aliquot (1 g) of the dried P. brevitarsis larvae powder pooled from three samples was suspended into 40 mL of distilled water and then boiled for 15 min. The treated solution was adjusted to 50 mL with distilled water. An aliquot (1 mL) of the treated solution was mixed with 1 mL of 5% trichloroacetic acid (TCA). The solution was mixed thoroughly using a vortex mixer (Genie 2, Scientific Industries, Bohemia, NY, USA), centrifuged for 10 min at 10,000×g, and the supernatant was filtered (0.25 μm Millipore filter). An aliquot (20 μL) of the filtered solution was examined using an amino acid analyzer (L-8900, Hitachi, Tokyo, Japan).
Total polyphenolic content was measured as described by Dewanto et al. (2002). Briefly, the sample was shaken three times for two hours with 80% methanol (SK-71 Shaker, JEIO Tech, Kimpo, Korea), filtered and concentrated under reduced pressure (N-1000, Eyela, Tokyo, Japan). Fifty microliter of each extract was mixed with 1 mL of 2% Na2CO3, the mixture was incubated for 3 min at room temperature, and then 50 uL of 50% Folin-Ciocalteu reagent was added (Sigma-Aldrich, St. Louis, MO, USA). The reaction was allowed to proceed for 30 min and then measured at 765 nm wavelength with a microplate reader (Multiscan GO, Thermo Fisher Scientific, Waltham, MA, USA). Total polyphenol content was presented as mg galic acid (GAE)/g equivalent.
Total flavonoid content was evaluated as described by Dewanto et al. (2002). Briefly, 250 μL of the extract was mixed with 1 mL of distilled water and 75 μL of 5% NaNO2, and then incubated for 5 min at room temperature. The solution was then mixed with 150 μL of 10% AlCl3·6H2O, incubated for 6 min at room temperature, followed by the addition of 500 μL of 1 N NaOH. The treated solution was incubated for 11 min at room temperature, and then measured at 510 nm wavelength with a microplate reader (Multiscan GO, Thermo Fisher Scientific). Total flavonoid content was presented as mg catechin/g equivalent.
Antioxidative activity of extracts were assessed by measuring ABTS (2,2'-azino-bis-3-ethylbenzo-thiazoline-6-sulfonic acid, Sigma-Aldrich) and DPPH (1,1-diphenyl-2-picrylhydrazyl, Sigma-Aldrich) radical scavenging activities (Lee and Lee, 2006). ABTS radical scavenging activity was measured as described by Re et al. (1999). ABTS was dissolved in water to a concentration of 7 mM. ABTS radical cation (ABTS·+) was obtained by incubation in the dark for 12–16 h at room temperature after mixing with 7 mM ABTS and 2.45 mM potassium persulfate. The reaction solution (50 uL of diluted samples and 1 mL of ABTS·+ solution) was incubated for 30 min at room temperature, and measured at 735 nm wavelength with a microplate reader Multiscan GO (Thermo Scientific Co. Ltd.). ABTS radical scavenging activity was presented as mg Trolox (TE)/g equivalent.
The reaction mixture for DPPH radical scavenging activity was composed of 0.8 mL of 0.2 mM DPPH and 0.2 mL of the extracts. These mixtures were incubated for 30 min at room temperature, and measured at 520 nm wavelength with a microplate reader Multiscan GO (Thermo Scientific Co. Ltd.). DPPH radical scavenging activity was presented as mg Trolox (TE)/g equivalent.
Results and Discussion
In order to establish rearing techniques capable of productively improving the quality of P. brevitarsis larvae and characterize the impact of various supplementary feeds on these larvae, the nutritional components were assessed following rearing with the supplementary listed in Table 1. Crude protein levels were highest (55.0%) in the control group; levels in the groups administered supplementary feeds were lowered to between 45.5% and 53.3%. It is known that the range of crude protein levels in P. brevitarsis larvae is 50.7%–57.9% and varies according to rearing conditions (Noh et al., 2015; Yeo et al., 2013). Here, all supplementary feeds tested lowered the protein content of P. brevitarsis larvae compared with the control group, a result which differs from previous studies (Song et al., 2017; van Borekhoven et al., 2015).
S. pumpkin, banana, S. persimmon, aloe and apple maintain crude protein levels of 1.5%, 1.0%, 0.5%, 0.05%, and 0.3%, respectively (data not shown). As a result of ingredient analysis by feed sources, crude protein contents in the larvae supplemented with S. pumpkin, banana and S. persimmon were lower compared with those supplemented with aloe and apple by 0.05% and 0.3%, respectively. It is presumed that crude protein content in the larvae are not affected by the relatively low protein content and high carbohydrate content of the supplementary feed sources in this study (van Borekhoven et al., 2015; Song et al., 2017). Therefore, we suggest that a more detailed study will be conducted in the future for further understanding changes in the nutritional components in P. brevitarsis larvae according to the protein source.
Crude fat content was 13.0% in the control group, and between 14.2% and 16.7% in the groups receiving supplementary feeds; the S. pumpkin group had the highest value among those receiving supplementary feeds. Crude fat content in P. brevitarsis larvae vary between 16.1% and 26.7% (Chung et al., 2013; Noh et al., 2015; Yeo et al., 2013). In this study, we note a crude fat content slightly lower than what has been previously reported. It is presumed that crude lipid content in the larvae affects owing to high carbohydrate content of the supplementary feed sources in this study.
In the groups receiving supplementary feeds, crude protein content decreased by 1.7%–9.5% when compared with the control group, whereas crude fat content increased by 1.2%–3.7%. The change in crude protein was reduced by as much as 9.5% (S. pumpkin) and as little as 1.7% (aloe). Increases in crude fat levels varied from 3.7% (S. pumpkin) to 1.2% (aloe). Assessments of crude protein and fat in this study indicated that these components are negatively correlated. Importantly, aloe demonstrated the highest potential as a supplementary food based on changes in the crude protein and fat levels compared with the control group (i.e., the smallest decrease in crude protein and the smallest increase crude fat levels).
Among the mineral components, phosphoric acid levels were reduced most significantly compared with the control group in larvae supplemented with S. pumpkin, whereas calcium, potassium, magnesium and sodium were decreased most significantly compared with the control group in those supplemented with S. persimmon. These results suggest that changes in the composition of minerals in P. brevitarsis are altered by supplementary feed.
Taken together, it is demonstrated here that changes in the composition of nutrients and minerals in P. brevitarsis larvae vary depending on the supplementary feed provided.
The fatty acid composition of larvae administered various supplementary feeds are presented in Fig. 1. Among them, oleic acid (C18:1) accounted for the largest amount (mean of 62.1%), followed by palmitic acid (C16:0) at 18.4%. The ratio of saturated:unsaturated fatty acids by total average (22.9:77.1) revealed that unsaturated fatty acids are 3.4 fold more abundant than saturated fatty acids (Table 2). The proportion of unsaturated fatty acids is lower than what was observed in a previous study (19.5:80.5; Yeo et al., 2013). The ratio of saturated:unsaturated fatty acids in the control group (24.6:75.4), revealed lower unsaturated fatty acid levels compared with the mean of the supplementary groups (Table 2). The ratio of saturated:unsaturated fatty acids varied from 20.3:79.7 to 22.1:77.9 in the groups administered supplementary feed, and the proportions of unsaturated fatty acids in the treated groups were increased by roughly 2.5% to 4.3% compared with the control group; the larvae receiving S. pumpkin demonstrated the highest fatty acid content (27.1:72.9, –2.5%). Therefore, the ratio of fatty acids varies when larvae receive supplementary feed; these changes vary depending on the type of supplementary feed provided.
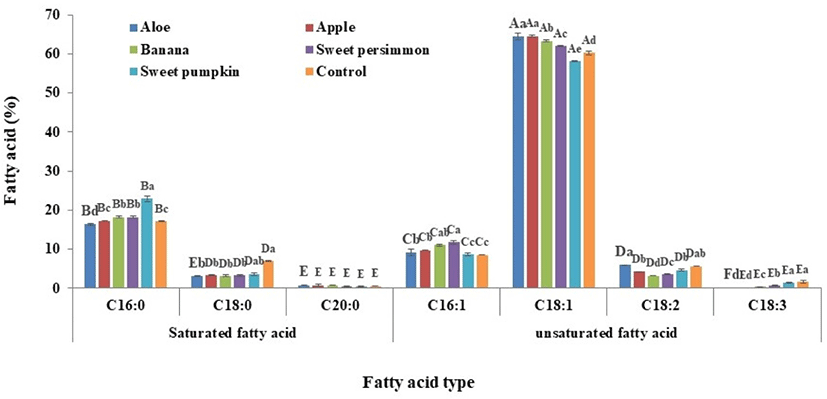
Supplementary feeds | Saturated fatty acid | Unsaturated fatty acid |
---|---|---|
Aloe | 20.3c1) | 79.7a |
Apple | 21.4c | 78.6a |
Banana | 22.1bc | 77.9ab |
Sweet persimmon | 22.0bc | 78.0ab |
Sweet pumpkin | 27.1a | 72.9c |
Control | 24.6b | 75.4b |
Oleic acid, an unsaturated fatty acid, increases the content of high density lipoprotein (HDL) in the blood but reduces the content of low density lipoprotein (LDL), and plays a critical role in the prevention of vascular disease through normalization of cholesterol levels (Chung et al., 2013; Nam and Lee, 2007; Zamora et al., 2001). Oleic acid is the most abundant fatty acid in this study and it generally increased compared with the control group in larvae receiving supplementary feed with the exception of those receiving S. pumpkin. These results demonstrate that: i) the ratio of fatty acids are altered to different extents depending on the source of supplementary feed, and ii) the derived products have a strong potential to serve as excellent functional foods.
A previous study showed that the composition of three edible mealworm species vary depending on the feed sources (van Borekhoven et al., 2015). As shown in Table 3, the total amino acid levels in larvae varied depending on the type of supplementary feed provided.
When compared with the control group, an increase in Met was only observed in the groups receiving supplemental aloe or apple and an increase in Phe was only observed in groups receiving supplemental apple or S. persimmon. The non-essential amino acids Pro and Cys were increased in those groups receiving supplemental banana or S. persimmon, and supplemental persimmon or S. pumpkin, respectively. The abundance of the remaining amino acids were the same or lower in the supplemental feed groups when compared with the control group.
The patterns of free amino acid levels varied depending on the supplementary feed provided (Table 4). Among the essential amino acids, the abundance of Met, Thr, Val, Leu, Ile, and Phe increased substantially in the group receiving supplemental aloe, however, the abundance of the remaining amino acids in the supplementary feed groups were similar or lower compared with the control group except for Lys and Phe which were increased in the groups receiving supplemental apple and S. pumpkin, respectively. These patterns were similar to what was observed when changes in total amino acids were assessed. Compared with the control group, the abundance of individual free non-essential amino acids were increased in the groups receiving supplemental aloe (Tyr), apple (Asp, Ser, and Gly), S. persimmon (Ser, Pro, and Tyr) and S. pumpkin (Asp, Ser, Pro, and Tyr). No increase in the level of free non-essential amino acids was observed in the group receiving supplemental banana. Additionally, the levels of the non-essential amino acids Glu, Ala, Cys, and Arg were not increased in any of the groups receiving supplemental feed. Although a similar pattern was observed in all treatment groups, we suggest that aloe has a strong possibility to serve as a useful supplementary feed since it was associated with a significant increase in non-essential amino acids.
Although the essential amino acids are indispensable for balance and growth of the body, they are necessarily supplied as food because the amino acids cannot be synthesized in the body. P. brevitarsis larvae maintain a large amount of these essential amino acids. Therefore, in order to induce an increase in more essential amino acids, it is suggested that various supplementary feeds be further tested and developed in the future.
Phenolic compounds vary in their structures and molecular weights, and the phenolic hydroxyl group of these compounds has antioxidant, anticancer and antibacterial activities via interaction with macromolecules such as proteins (Rice-Evans et al., 1997). Compared with the mean total polyphenol content of the control group (19.2 mg/g), the total polyphenol content of larvae receiving supplementary feeds was always higher; the combined mean from all groups receiving supplementary feed was 25.5 mg/g. Among the groups receiving supplemental feed, the highest and lowest polyphenol content was observed in those receiving S. pumpkin (30.4 mg/g) and S. persimmon (21.5 mg/g), respectively (Fig. 2A).
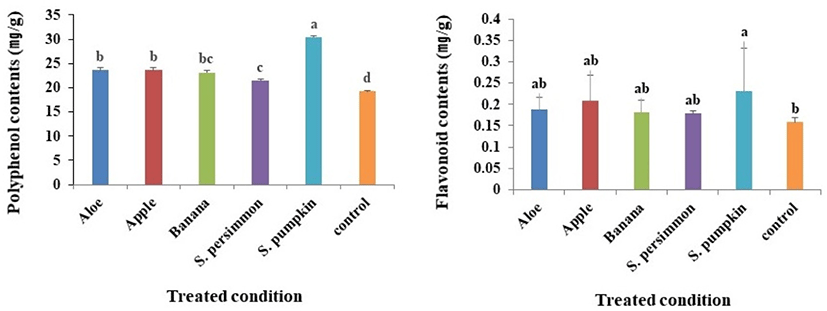
Flavonoids consist mainly of anthocyanidins, falvonols, flavones, cathechins and flavanones; specific flavonoids have a variety of physiological activities (eg, antioxidation, antibacterial) depending on their structure (Camargo et al., 2017; Middleton and Kandaswami, 1994). Total flavonoid content of P. brevitarsis larvae varied depending on the source of supplementary feed and significant changes were observed when comparing those receiving supplementary feed with the control group and previously described polyphenol contents (Fig. 2B). Total flavonoid contents in the group receiving supplemental S. pumpkin was 0.23 mg/g. Although the total flavonoid contents in groups receiving supplemental aloe, apple, banana and S. persimmon were higher compared with the control group, these differences were not significant.
Antioxidant materials of natural products donate electrons to active radicals and suppress the oxidation of fat in foods. Therefore, in humans these materials play critical roles by suppressing aging mediated by active radicals (Lee and Lee, 2006; Lee et al., 2017b; Xie and Schaich, 2014; Yoon et al., 2007). ABTS radical scavenging activity varied depending on the supplementary feed provided and some of these differences were substantial. The ABTS activity of P. brevitarsis larvae in the control group was the lowest (13.5 mg/TE g), and although all the groups receiving supplementary feed had significantly higher levels compared with the control group, the differences among the treated groups were not statistically significant (Fig. 3A).
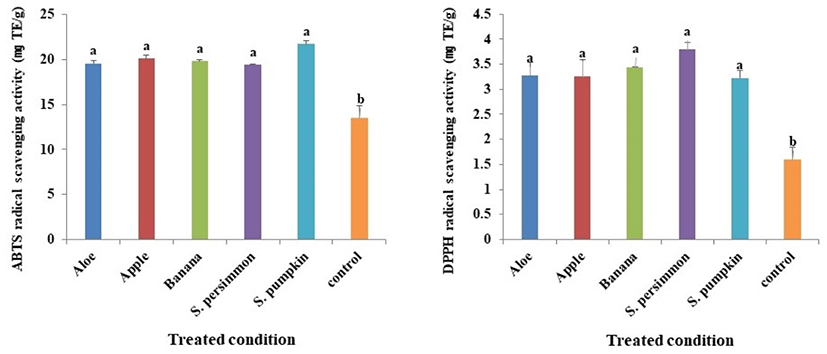
DPPH radical scavenging activity varied depending on the supplementary feed provided in a pattern similar to what was observed for ABTS radical scavenging activity as described above. The activity levels were significantly different between the treated and control groups, but not among the treated groups (Fig. 3B).
Since antioxidative activities in the groups supplied with supplementary feeds were higher compared with the control group, it is assumed that supplementary feeds employed in this study can be utilized to enhance function of P. brevitarsis larvae. Finally, we suggest that not only can functional improvements in P. brevitarsis larvae be achieved by providing them with certain supplementary feeds, but also that their capacity to serve as a healthy functional food can be altered depending on the breeding environment.
Conclusion
In P. brevitarsis larvae, supplementary feeds led to decreased abundance of crude protein and increased abundance of unsaturated fatty acids. The most abundant of all fatty acids was oleic acid (58.2%–64.5%), and the high levels of unsaturated fatty acids were maintained (22.9:77.1% saturated:unsaturated fatty acids). When compared with the control group, antioxidant substances and antioxidant activity were greatly increased in larvae administered supplementary feeds. In P. brevitarsis larvae, supplementary feeds led to: i) decreased levels of protein, ii) increased levels of unsaturated fatty acids and iii) increased antioxidative capacity.