Introduction
The skin is composed of a highly distinct, multilayered epithelium, which contains approximately 30% of the total water content of the body (Madison, 2003; Sougrat et al., 2002). The stratum corneum, the outermost layer of the skin, serves as the primary line of defense against the external environment, and maintains homeostasis by regulating the evaporation of water (Jacobi, 1959). The moisture present in the stratum corneum contains various physiologically active substances that are necessary for the human body and help the skin remain smooth and moist; this is important for the healing of various types of skin damage (Greaves and Søndergaard, 1970; Verkman et al., 2008). A failure in the ability to maintain water homeostasis in the skin results in various skin diseases, such as atopic dermatitis (Sator et al., 2003; Segre, 2006), psoriasis (Ghadially et al., 1996), and wound-healing delay (Gallant‐Behm and Mustoe, 2010).
Aquaporin (AQP) is a small, hydrophobic membrane protein that transports water molecules into cells, and thus regulates the process of water retention in cells (Agre et al., 2002; Hara-Chikuma and Verkman, 2008a). Till date, 13 mammalian AQPs (AQP-0 to AQP-12) have been cloned; these can be classified into different categories, depending on their transport capacity. AQP-0, AQP-1, AQP-2, AQP-4, and AQP-5 selectively transport water; however, AQP-3, AQP-7, AQP-9, and AQP-10 transport water and small solutes (such as glycerol) (Agre et al., 2002; Verkman, 2011). Several types of AQPs are reportedly present in skin and skin appendages. Among these, AQP-3 forms the water and glycerol channels present in the basal layer keratinocytes of the skin epidermis (Agre et al., 2002; Hara-Chikuma and Verkman, 2005), and is crucial for the process of skin moisturization (Hara et al., 2002; Ma et al., 2002; Sougrat et al., 2002). In addition, AQP-3 is important for wound healing, as it promotes epithelial cell migration and proliferation (Hara et al., 2002; Hara-Chikuma and Verkman, 2008b; Verkman et al., 2008). AQP-3 knockout mice show severe skin disorders, such as impaired skin hydration, decreased wound-healing ability, and reduced skin elasticity (Hara et al., 2002; Ma et al., 2002). Therefore, the stimulation of AQP-3 expression in keratinocytes provides effective levels of epidermal hydration and could be used to effectively improve the procedures used for skin disease treatment.
Whey is produced as a by-product of the rennet-type cheese manufacturing process and is collected after the coagulation of casein and fat in fermented milk. Whey accounts for 85%–90% of the total milk volume and retains soluble whey proteins, growth factors, lactose, and minerals, along with microbial fermentation metabolites (Smithers, 2008; Yadav et al., 2015). Furthermore, the components of whey regulate the differentiation of cultured human epidermal keratinocytes, while whey peptides improve the wound-healing process in the skin (Baba et al., 2006; Wang et al., 2010).
Colostrum refers to the milk secreted within 72 h of delivery (Thapa, 2005). Colostrum is composed of three primary components: nutrients (Macy, 1949), immune substances (Ogra et al., 1977), and growth factors (Pakkanen and Aalto, 1997; Seo et al., 2018). Functionally, colostrum is known to enhance immune function and eliminate bacteria, toxins, and allergens (Zimecki and Kruzel, 2007). Notably, the growth factors in the colostrum play an important role in wound-healing by promoting keratinocyte and fibroblast proliferation and migration (Chen et al., 2014; Hara and Verkman, 2002; Kovacs et al., 2009).
Despite the beneficial functions of colostrum, only a few studies have attempted to determine the effect of colostrum on the skin or identify the mechanism underlying colostrum-induced keratinocyte proliferation. In the present study, we used HaCaT cells to investigate the relationship between colostrum and AQP-3 expression, which plays an essential role in keratinocyte proliferation.
Materials and Methods
Lactobacillus bulgaricus (KCTC 3635) and Streptococcus thermophilus (KCTC 3658) were obtained from the Korean Collection for Type Cultures (KCTC), and L. rhamnosus GG (ATCC 53103) was purchased from the American Type Culture Collection (ATCC, Manassas, VA, USA). The three bacterial strains were cultured for 24 h at 37°C in MRS broth (Difco, Becton, Dickinson and Company, Sparks, MD, USA), transferred to fresh MRS broth, and incubated overnight at 37°C. The activated cells were harvested via centrifugation at 850×g for 10 min at 4°C, washed twice, and then resuspended in phosphate-buffered saline (PBS). A starter culture was inoculated into the defatted colostrum and milk samples.
The milk and colostrum used in this study were obtained from the Cheongwon pasture in Chungbuk, Korea. Colostrum was collected within 24 h after calf birth, whereas milk was collected randomly. Both colostrum and milk were pasteurized at 63°C for 30 min and centrifuged at 3,000×g at 4°C for 30 min to remove cellular debris and fat. Fermentations were performed in sealed bottles containing 500 mL of the fat-free milk and colostrum with three kinds of bacterial strains, which included L. bulgaricus (KCTC 3635), S. thermophilus (KCTC 3658), and L. rhamnosus GG (ATCC 53103), for 1 h at 37°C in an incubator. After fermented samples were incubated with rennet (0.2 μL/mL) at 37°C for 30 min, they were centrifuged at 10,000×g for 1 h at 4°C for separation into a solid (curd) and a liquid (whey) layer. Finally, the whey samples were filtered using a 0.45 μm syringe filter (Sartorius Stedim Biotech, Goettingen, Germany), lyophilized, and stored at –20°C until further use. The final lyophilized product was dissolved in water to obtain a solution with an appropriate concentration. Colostrum whey, milk whey, fermented colostrum whey, and fermented milk whey samples were used for further experiments.
The human skin keratinocyte cell line HaCaT (KCLB, Seoul, Korea) was maintained in Dulbecco’s Modified Eagle’s Medium (Welgene, Gyeongsan, Korea) supplemented with 10% fetal bovine serum (Welgene) and 1% penicillin-streptomycin (Lonza, Walkersville, MD, USA) at 37°C under 5% CO2 conditions. In addition, Du145 cells were maintained in RPMI 1640 medium (Welgene) supplemented with L-glutamine (300 mg/L), 25 mM HEPES, 25 mM NaHCO3, and 10% fetal bovine serum (Welgene). The HaCaT and Du145 cells were sub-cultured once every three days. Dulbecco’s PBS (DPBS; Welgene) and 0.25% trypsin-EDTA (Thermo Fisher Scientific, Rockford, IL, USA) were used also used during cell culture.
The cell viability assay was performed using an EZ-Cytox cell viability assay kit (EZ-3000, DoGen, Seoul, Korea). HaCaT cells were seeded into 12-well plates (approximately 3×104 cells/well) and treated with 0, 1, 10, 100, 200, and 400 μg/mL of the colostrum whey, milk whey, fermented colostrum whey, and fermented milk whey samples for 24 h. Subsequently, 100 μL of EZ-Cytox reagent was added to each well and incubation was performed for 1 h. Two-hundred microliters of the supernatant were transferred into each well of a 96-well plate, and the absorbance of the samples was measured at 450 nm using a microplate reader (Tecan Sunrise, Salzburg, Austria). Cell viability values were normalized to those of the cells from the untreated control groups.
Total RNA was isolated from HaCaT cells using the RNeasy Mini Kit (Qiagen, Hilden, Germany), along with on-column DNase treatment (Qiagen), as per the manufacturer’s instructions. A cDNA synthesis kit (MGmed, Seoul, Korea) was used to synthesize cDNA from 1.5 μg of total RNA, using the Oligo (dT) 30 primer. Target gene expression was analyzed using the gene-specific primers listed in Table 1. The amplified products were subjected to electrophoresis on a 1.5% agarose gel and visualized with ultraviolet illumination.
RT-PCR was performed at 94°C for 2 min, followed by 35 cycles at 94°C for 10 s, 60°C for 30 s, and 72°C for 20 s. For QPCR, cDNA strands synthesized from the isolated total RNA were used as templates. PCR was performed on a StepOnePlus Real-Time PCR System (Qiagen), using the SYBR Green PCR Mastermix (BioRad, Hercules, CA, USA). The cycle threshold values were normalized against the GAPDH gene expression levels. PCR was performed at 94°C for 1 min; subsequently, 40 cycles were performed at 94°C for 10 s, 57°C for 10 s, and 72°C for 20 s.
Treated and untreated cultured HaCaT cells were harvested with radioimmunoprecipitation assay (RIPA) buffer supplemented with a protease inhibitor. The total protein content was quantified using the BCA Protein Assay (Thermo Scientific, Seoul, Korea). Equal quantities of proteins (40 μg each) were separated using 12% polyacrylamide gel electrophoresis, and the resultant bands were transferred onto polyvinylidene difluoride membranes. Non-specific binding was blocked using TBST containing 1% bovine serum albumin (BSA) at 26°C for 1 h. Subsequently, the membrane was incubated overnight at 4°C with primary antibodies diluted in TBST and a solution containing 1% BSA: AQP-3 (1:2,000 dilution; Merck Millipore, MA, USA), phospho-extracellular-signal-regulated kinase (ERK) (1:2,000 dilution; Cell Signaling Technology, Beverly, MA, USA), ERK (1:1,000 dilution; Cell Signaling Technology), phospho-Jun N-terminal kinase (JNK) (1:1,000 dilution; Cell Signaling Technology), JNK (1:1,000 dilution; Cell Signaling Technology), phospho-p38 (1:1,000 dilution; Cell Signaling Technology), p38 (1:1,000 dilution; Cell Signaling Technology), and β-actin (1:1,000 dilution; Santa Cruz Biotechnology, Santa Cruz, CA). The membranes were then washed in TBST and incubated at room temperature (RT) for 1 h with horseradish peroxidase-linked anti-rabbit or anti-mouse IgGs (1:10,000 dilution; Santa Cruz Biotechnology, Santa Cruz, CA). Proteins were visualized using an enhanced chemiluminescence detection system (Thermo Scientific) and autoradiography X-ray film (AGFA, Mortsel, Belgium). β-actin was used as a control to determine if each sample contained the same amount of protein.
To detect cellular AQP-3 membrane protein, HaCaT cells were seeded (approximately 1×105 cells/well) into a 6-well plate and treated with varying concentrations (0, 1, 10, and 100 μg/mL) of fermented colostrum whey for 3 h. HaCaT cells were rinsed with DPBS, fixed with 4% paraformaldehyde for 15 min; then, membrane permeabilization was performed for 10 min with PBS containing 0.2% Triton X-100. Non-specific protein binding was blocked using 1% BSA in PBS for 30 min at RT, and the cells were incubated overnight at 4°C with AQP-3 primary antibodies (Millipore, diluted 1:200) in 1% BSA in DPBS. The cells were then washed three times with DPBS and incubated with secondary goat anti-rabbit IgG antibodies (1:1,000 dilution; Santa Cruz Biotechnology, Texas, USA) for 1 h at RT in 1% BSA in DPBS. Samples were incubated with 3’-diaminobenzidine (DAB) solution (Burlingame, CA, USA) prepared in DPBS for 5 min for DAB staining. Finally, the samples were mounted with a mounting solution (DAKO, Carpinteria, CA, USA).
The SPSS software (version 24.0) for Windows (SPSS, Chicago, Il, USA) was used for the statistical analysis. Differences among groups or time points were determined using one-way analysis of variance, followed by Tukey’s honest significant difference test. Different significance levels have been indicated using different lowercase letters.
Results and Discussion
The cell viability assay was performed to investigate the cytotoxicity of colostrum whey, milk, fermented colostrum, and fermented milk in HaCaT cells. HaCaT cells were treated with increasing concentrations of colostrum, milk, fermented colostrum, and fermented milk whey (1, 10, 100, 200, and 400 μg/mL) for 24 h; the corresponding viabilities of HaCaT cells treated with these varied concentrations of colostrum whey were 102.19±1.06%, 101.13±1.08%, 104.16±0.87%, 104.41±1.68%, and 107.48±1.09%, respectively. Values were expressed as a percent of the control group values (regarded as 100%). The viabilities of milk whey (1, 10, 100, 200, and 400 μg/mL)-treated cells were 96.28±1.07%, 98.27±1.25%, 98.98±2.23%, 103.70±1.08%, and 107.51±0.59%, respectively; the viabilities of the fermented colostrum whey (1, 10, 100, 200, and 400 μg/mL)-treated cells were 101.72±1.27%, 104.31±0.97%, 108.97±1.34%, 123.17±1.08%, and 124.49±1.94%, respectively; and those of the fermented milk whey (1, 10, 100, 200, and 400 μg/mL)-treated cells were 104.95±1.39%, 105.52±2.71%, 106.55±1.21%, 107.23±0.57%, and 112.92±0.37%, respectively (Fig. 1A). No notable cytotoxicity was observed in all treated HaCaT cell samples at concentrations of up to 400 μg/mL. The cell viability increased significantly at a concentration of 400 μg/mL in case of all samples; especially, the viability increased significantly from 100 μg/mL to 400 μg/mL in case of the cells treated with fermented colostrum whey (Fig. 1A). These results indicated that fermented colostrum whey significantly increased cellular proliferation. Yoon et al. (2002) reported that treatment of keratinocytes with bovine colostrum promoted keratinocyte proliferation. In addition, bovine colostrum promoted proliferation of HaCaT cell, although no significant changes were seen in HaCaT cell growth after 48 h of colostrum treatment, which suggests that the enriched bioactive substances in the colostrum plays an essential role in HaCaT cell proliferation (Kovacs et al., 2009). These previous studies and the current data together reveal the ability of colostrum to promote HaCaT cell proliferation.
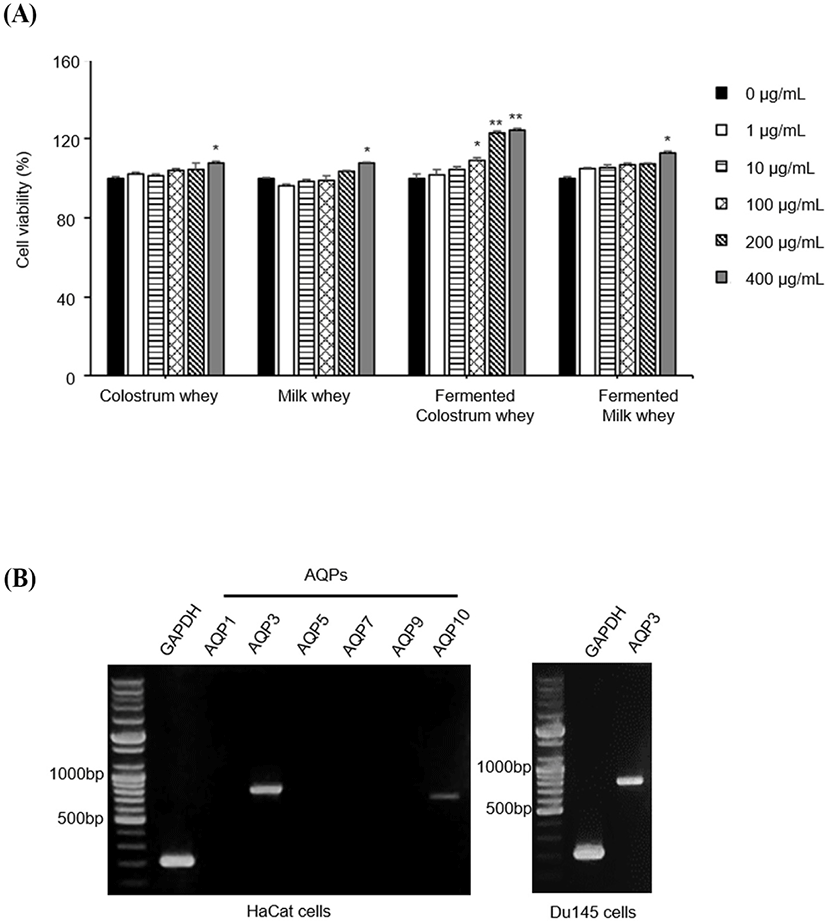
To identify the subtypes of AQPs expressed in HaCaT, we performed RT-PCR using AQP-specific primers (Table 1). Among the tested AQPs, the expression levels of AQP-3 and AQP-10 mRNAs were identified; AQP-3 expression was abundant in HaCaT cells and was observed to occur in DU145 cells, which were used as positive controls (Fig. 1B). DU145 cells are prostate cancer cell line, and it is well known that AQP3 is expressed in these cells (de Almeida et al., 2021). In the correlation of keratinocyte proliferation with AQP-3 expression, two complex mechanisms were found to be defined and undefined, respectively. AQP-3-mediated cell proliferation is one of the well-defined mechanisms. Human keratinocytes with AQP-3 knockdown and keratinocytes from AQP-3-knockout mice showed reduced levels of proliferation (Hara-Chikuma and Verkman, 2008a). The upregulation of AQP-3 by the transfection of AQP-3 plasmid DNA has been shown to promote the proliferation of human keratinocytes and increased cellular glycerol and ATP levels (Nakahigashi et al., 2011).
Next, we investigated whether the whey obtained from colostrum, milk, fermented colostrum, and fermented milk altered the expression of AQP-3. HaCaT cells were treated with 1, 10, or 100 μg/mL of all four whey samples, and the AQP-3 mRNA level was measured using RT-PCR and QPCR (Fig. 2). AQP-3 mRNA expression was not altered in cells treated with colostrum, milk, and fermented milk (Fig. 2A, B and D, respectively), but was markedly increased in cells treated with fermented colostrum whey at a concentration of 100 μg/mL (Fig. 2C). Consistently, the QPCR analysis data showed that treatment with colostrum and milk did not alter the AQP-3 expression at any concentration, but treatment with fermented colostrum significantly increased the AQP-3 expression at 10 and 100 μg/mL (Fig. 2E); the increase in the AQP-3 expression level was 3.06±0.05-fold higher than that observed for cells treated with milk or colostrum (at a concentration of 100 μg/mL, Fig. 2E). In addition, treatment with fermented milk resulted in a significant 1.23±0.05-fold increase in the AQP-3 expression level at a concentration of 100 μg/mL compared with the level observed with milk and colostrum (Fig. 2E). The duration of AQP-3 expression after treatment with fermented colostrum was determined by treating HaCaT cells with 100 μg/mL of fermented colostrum. The expression of AQP-3 mRNA increased after treating cells for 2 h, peaked at 3 h, and decreased after 6 h (Fig. 2F).
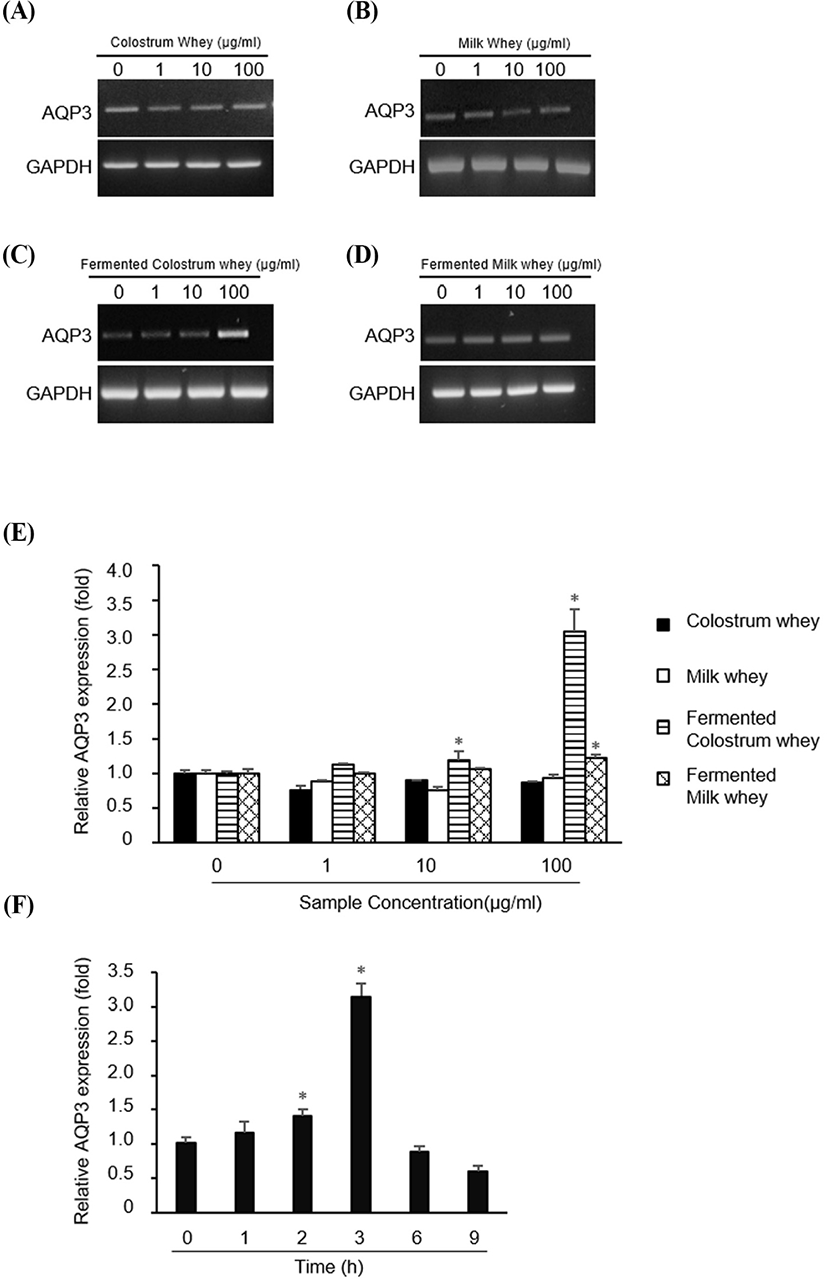
To identify AQP-3 expression at the protein level (Fig. 3), HaCaT cells were treated with 0, 1, 10, and 100 μg/mL of fermented colostrum for 3 h, and subsequently analyzed via western blotting and immunocytochemistry. AQP-3 protein expression levels increased following fermented colostrum treatment at concentrations of 10 and 100 μg/mL (Fig. 3A). Densitometric analyses of AQP-3 and β-actin ismmunoblots showed that AQP-3 protein expression levels were 10.5 and 10.9-fold higher than that of the negative control (Fig. 3B). Consistently, the immunocytochemistry data also showed that AQP-3 was expressed in the cell membrane, and strong AQP-3 staining signal was visually observed in fermented colostrum-treated cells compared with that in the control in a dose-dependent manner (Fig. 3C). These data demonstrate that the upregulation of AQP-3 expression in keratinocyte occurred because of fermented colostrum treatment.
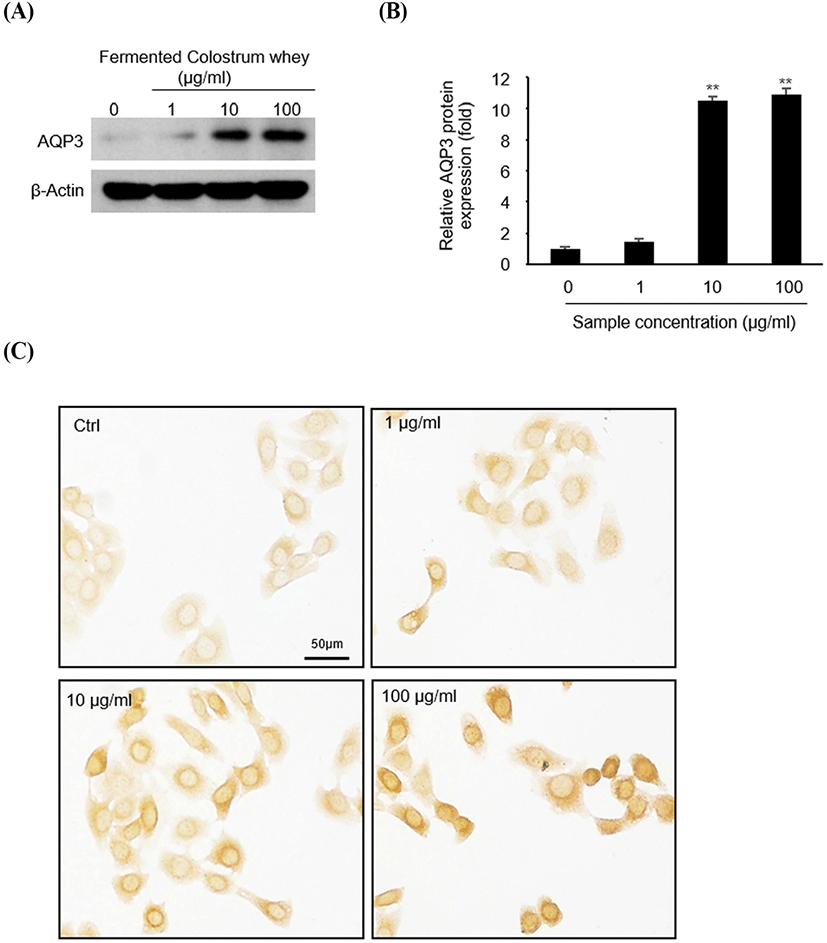
Currently, many studies are investigating the active compounds that increase AQP-3 expression levels, to enable the treatment of skin disorders. Many studies have reported plant-derived substances such as green Coffee arabica seed oil extract (Del Carmen Velazquez Pereda et al., 2009), trans-zeatin purified from Zea mays (Yang et al., 2009), Piptadenia colubrina extract (Del Carmen Velazquez Pereda et al., 2009), and asiaticoside, isolated from Centella asiatica (Wijayadi and Darmawan, 2017).
Although there is no direct evidence in this study, it is possible that fermented colostrum regulates HaCaT keratinocyte proliferation via the upregulation of AQP-3. EGF, which is abundantly present in colostrum, is a known upstream regulator of AQP-3 expression in cultured colorectal cancer (Li et al., 2013), ovarian cancer (Ji et al., 2008), and pancreatic cancer (Liu et al., 2012) cells. As expected, the upregulation of AQP-3 in cancer cells resulted in their enhanced proliferation. Milk and fermented milk whey contain various bioactive substances that regulate skin cells. For instance, lactoferrin stimulates proliferation and wound healing in epidermal keratinocytes (Tang et al., 2010), and exopolysaccharides from Streptococcus thermophilus prevent the occurrence of ultraviolet-induced skin damage (Morifuji et al., 2017). Additionally, bioactive peptides with low molecular mass are also produced during milk fermentation and the rennet enzyme reaction (Shinagawa et al., 2018). Shinagawa et al. (2018) reported the increase in keratinocyte proliferation with increase in AQP-3 expression after treatment with compounds with molecular weight <3 kDa that were produced during the cheese fermentation process. Interestingly, lactose, calcium, and other abundant molecules with low molecular mass did not affect AQP-3 expression and keratinocyte proliferation (Shinagawa et al., 2018), suggesting that low-molecular-weight peptides produced during fermentation activated the keratinocytes. A previous study found that milk whey was abundantly present in phospholipids, which supports this hypothesis (Vaghela and Kilara, 1995). The topical treatment of murine dorsal skin with phospholipids promotes keratinocyte proliferation and differentiation (Kumura et al., 2012). Fermented colostrum whey might contain abundant amounts of both bioactive peptides and phospholipids that were generated by fermentation. Because we found that the use of fermented colostrum whey resulted in 2-fold higher AQP-3 expression levels than those following treatment with fermented milk whey, it is possible that fermented colostrum whey has a greater abundance of bioactive peptides associated with AQP-3 expression than fermented milk whey. However, the specific whey components that regulate AQP-3 expression in HaCaT keratinocytes remain unclear. Further experiments are required to understand the regulatory effects of fermented colostrum whey components on HaCaT keratinocytes.
Interestingly, AQP-3 expression levels were maximal at 3 h post treatment with fermented colostrum whey and decreased to levels equivalent to those of the control within 6 h. Other studies also showed that the change in AQP-3 expression caused by external stimuli occurs rapidly. For examples, when cells were treated with 10–20 mg/mL of Piptadenia colubrina extract, AQP-3 expression increased after 2 h, peaked at 6 h, and decreased after 24 h (Del Carmen Velazquez Pereda et al., 2010). Treatment with a green Coffea arabica seed extract resulted in increased AQP-3 expression after 3 to 6 h (Del Carmen Velazquez Pereda et al., 2009). These results support our observation that the AQP-3 expression level began to increase after 2 h and peaked at 3 h.
To understand the regulatory mechanism by which fermented colostrum whey effectively induced HaCaT cell proliferation, the present study investigated whether mitogen-activated protein kinases (MAPK) pathways were involved in the proliferation of HaCaT cells, using fermented colostrum whey. The phosphorylation levels of three MAPKs, including ERK 1/2, JNK1/2, and p38, were assessed. HaCaT keratinocytes were treated with 100 μg/mL of fermented colostrum whey for varying durations. The results showed that treatment with 100 μg/mL of fermented colostrum whey rapidly induced the phosphorylation of JNK1/2 and p38 MAPK within 20 min (Fig 4A and B), whereas the levels of phosphorylated ERK 1/2 were not increased (Fig. 4C). In summary, exposure to fermented colostrum whey increased the expression level of AQP-3 mRNA in, and induced the proliferation of, HaCaT keratinocytes via the activation of p38 and pJNK signaling. In the present study, the phosphorylation of p38 MAPK and JNK were observed in HaCaT keratinocytes after their treatment with fermented colostrum whey. Although studies identifying the underlying mechanism of keratinocyte proliferation and effects of colostrum or milk have not been undertaken thus far, similar results were seen with human osteoblasts. The treatment of human osteoblasts with concentrated low-molecular-weight bovine milk whey proteins (1–30 kDa) increased alkaline phosphatase activity and mineralization, by upregulating JNK-activating transcription factor 4 (ATF4) via the phosphorylation of JNK (Tsuji-Naito and Jack, 2012). Interestingly, they used low-molecular-weight (1–30 kDa) fractions of whey proteins, and this fraction typically exhibited an abundance of growth factors and cytokines (Hwang et al., 2012).
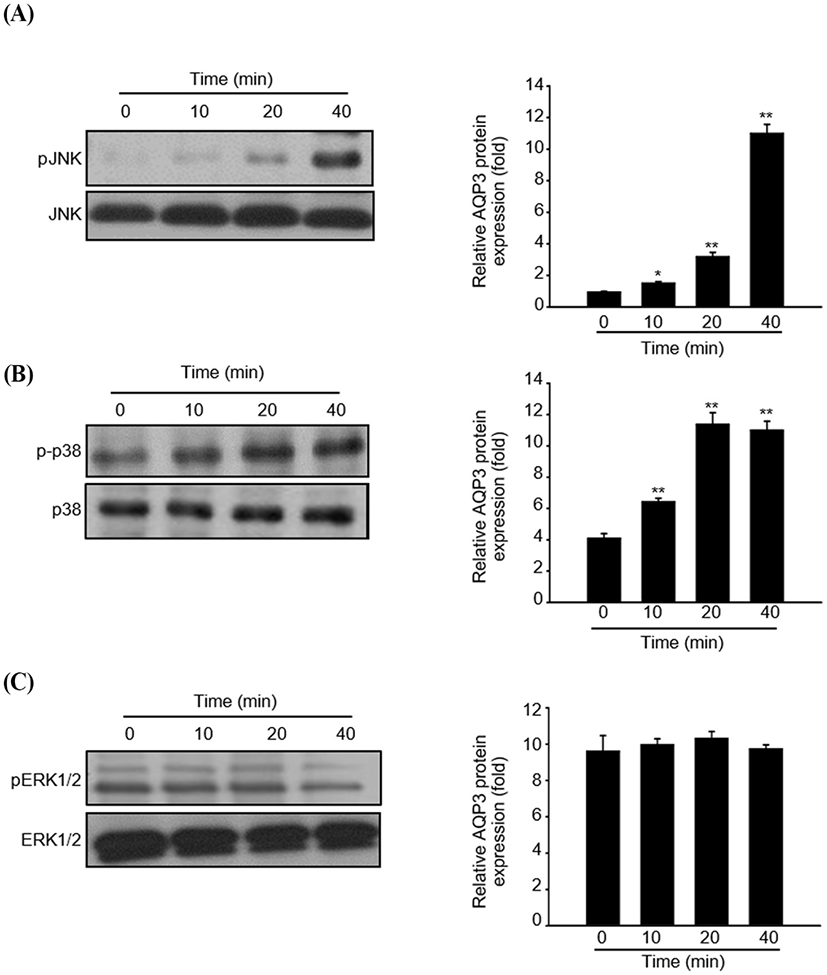
These prior reports and our data provide strong evidence that certain factors in the colostrum are important for keratinocyte activation. MAPK families play a crucial role in the regulation of different processes, including cell proliferation, development, differentiation, transformation, and apoptosis. Five distinct groups of MAPKs have been characterized in mammals, including ERK1/2, JNKs, p38, and ERK3, 4, and 5. In addition, the most extensively studied groups of vertebrate MAPKs include ERK1/2, JNKs, and p38 kinases (Kyriakis and Avruch, 2001). Evidence has shown that the JNK signal pathway was involved in keratinocyte proliferation. Gazel et al. (2006) has reported about JNK-induced epidermal keratinocyte proliferation and the inhibition of differentiation. In addition, p38 MAPKs were defined as cellular protein kinases that were activated in response to stresses, such as osmotic stress, inflammatory cytokines, ultraviolet light, and changes in the oxygen content (Brewster et al., 1993; Han et al., 1994). In contrast, a recent study has found that p38-MAPKs were also activated in response to a variety of extracellular growth and differentiation cues (Nebreda and Porras, 2000). Although ERK1/2 was not activated, fermented colostrum whey induced HaCaT cell proliferation and also the activation of the p38 MAPK and JNK signaling pathways (Fig. 5). In contrast with our results, Li et al. (2015) reported the downregulation of AQP-3 expression by lipopolysaccharides via the p38/c-Jun signaling pathway in HT-29 human colon epithelial cells. Taken together, our results suggest that fermented colostrum whey has potential for effectively improving the efficacy of treatment procedures for various skin disorders, as it activates AQP-3 expression and proliferation with activation of p38/c-Jun N-terminal kinase in keratinocytes.
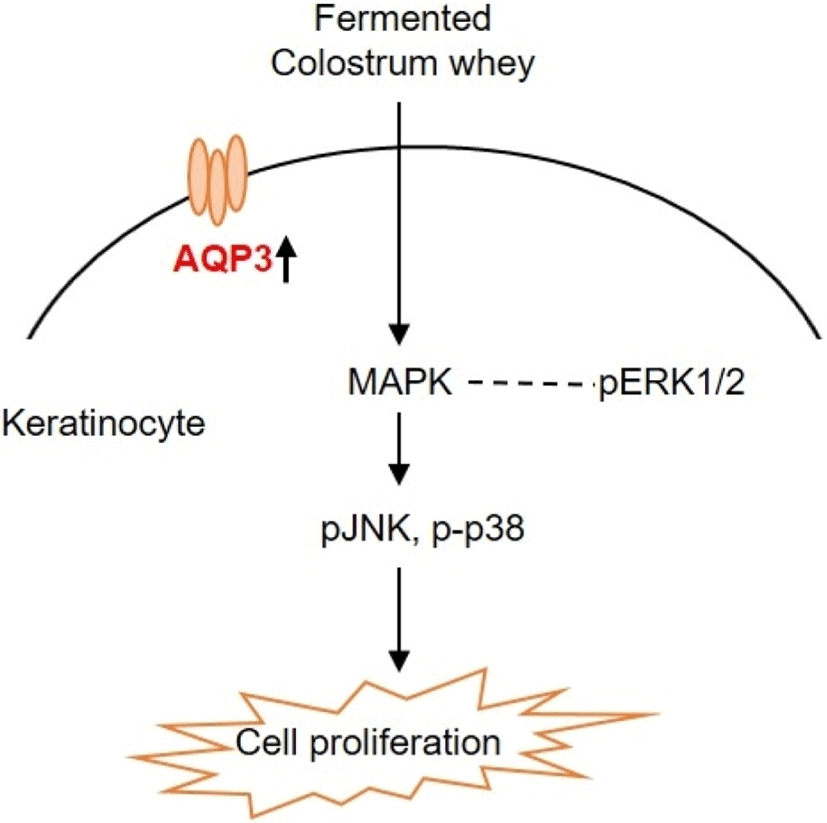