Introduction
Carbohydrate percentage ranges from a trace to over 10% with lactose as a predominant component in the colostrum or milk of mammals. Other carbohydrate components are constituted by different type of sugars commonly known as milk oligosaccharides (MOS) (Urashima and Taufik, 2010). Oligosaccharides are sugars which are normally composed of 3 to 15 monosaccharides covalently bound by glycosidic linkages. MOS are mostly composed of a lactose core plus hexoses, deoxyhexoses, N-acetylhexosamines, neuraminic acid and fucose (Sundekilde et al., 2012). Usually, in the eutherian species, lactose is a major component over MOS, while in the monotremes, marsupials and some Carnivore species, MOS predominate over lactose (Urashima and Taufik, 2010; Urashima et al., 2013). Colostrum and mature human milk contain 22−24 and 12−13 g/L of human milk oligosaccharides (hMOS), respectively; this oligosaccharide fraction is the third largest solid component, after lactose and lipid (Newburg and Neubauer, 1995). Almost all MOS are having lactose unit in their reducing end, they are divided into two groups; the neutral ones, which do not contain any charged monosaccharide residues, and the acidic ones, which contain one or more negatively charged residues of sialic acid (Gopal and Gill, 2000).
Lactose is generally recognized as an energy source for the newborns (Urashima et al., 2001), while MOS have been observed to have important roles in the human small intestine, and they also affect significantly on the immune and nervous system developments in neonates (Bode, 2012; Plaza-Díaz et al., 2018). Oligosaccharides may act as epithelial receptors for pathogens, thus acting as a potential protector of mucosal cells (Bode, 2012; Bode and Jantscher-Krenn, 2012; Coppa et al., 2006; Crane et al., 1994; Kunz et al., 2014; Lane et al., 2010). Due to these bioactive functions, MOS are thought to have potential on treating bacterial infections and inflammatory (Daddaoua et al., 2006; Lane et al., 2012; Newburg, 1999; Newburg, 2000; Newburg et al., 2016; Perret et al., 2005; Ruiz-Palacios et al., 2003; Yue et al., 2020; Zopf and Roth, 1996).
Human milk is the gold standard for oligosaccharide structure variability and benefit. However, due to ethical and technical constraints, these compounds of human/breast milk cannot be collected on a large amount to evaluate their biological activities in vitro and in vivo as well as for the purpose of infant formula supplementation (Oliveira et al., 2015). Therefore, the colostrum or milk of dairy animals and their products could be used as alternative sources. Oligosaccharides derived from these sources can be utilized by industry for the development of functional foods in the clinical and infant or even adult nutrition (Sousa et al., 2019a).
Heterogeneity of oligosaccharides was observed among animals. Several studies have been done to characterize MOS from colostrum and milk of dairy animals such as: cows (Albrecht et al., 2014; Barile et al., 2010; Nakamura et al., 2003; Saito et al., 1984; Saito et al., 1987), goats (Albrecht et al., 2014; Chatziioannou et al., 2021; Claps et al., 2014; Claps et al., 2016; Lu et al., 2020; Martín-Ortiz et al., 2016; Martín-Ortiz et al., 2017; Martinez-Ferez et al., 2006a; Martinez-Ferez et al., 2006b; Urashima et al., 1994; Urashima et al., 1997; Viverge et al., 1997), sheeps (Nakamura et al., 1998; Sasaki et al., 2016), camels (Alhaj et al., 2013; Fukuda et al., 2010), and reindeer (Taufik et al., 2014). MOS content is varied in the milk or colostrum of different dairy species. Quantitative studies of goat milk oligosaccharides (gMOS) reported a content ranging from 60 to 350 mg/L in mature milk and from 200 to 650 mg/L in colostrum. These numbers are much lower than in human milk/colostrum but higher than that of other domesticated animals, e.g., cows (bMOS; 30−60 mg/L) and sheeps (sMOS; 20−40 mg/L) (van Leeuwen et al., 2020).
The above-mentioned MOS studies on Capra hircus (goat) milk and colostrum were mostly used for the well-known breed of dairy goat such as Alpine and Saanen, only limited of them using local breed such as Guanzhong and Shandong (China), Garganica and Maltese (Italy) and Murciano-Granadina (Spain). However, no studies on the characterization of MOS from Indonesia dairy animals, including goat have been done so far.
In Indonesia, goat is the second in terms of milk production and consumption after cow milk. There are three dairy goat breeds reared mostly by local farmers, namely: Saanen, Etawah Grade (crossbreed of a local goat with the Etawah breed from India) and Sapera (crossbreed of Saanen from Europe with Etawah Grade). Among those breeds, the most reared breed is Etawah Grade. Indonesia ranks first in the Southeast Asia region (Association of Southeast Asian Nations, ASEAN) for goat milk production. According to the Food and Agriculture Organization (FAO, 2021), in 2019 Indonesia produced 369,584 tons of fresh whole goat milk with a total goat population (dairy and meat type) of 19,096,381 heads in 2020 [Badan Pusat Statistik (BPS), 2021]. This is an abundant and promising alternative source for harvesting MOS from natural resources to be further utilized for the food or pharmaceutical industries. Therefore, the present study investigated for the first time the profile of MOS, especially sialylated or sialyl oligosaccharides (SOS) from Etawah Grade colostrum (EGC).
Materials and Methods
Colostrum samples were collected from two dairy goat farms (Farms I and II) located in Cijeruk and Leuwiliang sub-districts, Bogor district, West Java province, Indonesia. Colostrum samples from Farms I and II were pooled from three to four heads of Etawah Grade goats, respectively. Both samples were collected within 24 hours post-partum. The samples were then labelled as EGCI and EGCII and immediately stored at −20°C until use. Oligosaccharide reference materials (see Table 1 for abbreviations) 3’-SL and 6’-SL were obtained from Sigma-Aldrich (St. Louis, MO, USA). 3’-Neu5GCL was isolated from Greater Galago milk (Taufik et al., 2012), 6’-NeuGcL was isolated from caprine and ovine colostrum (Nakamura et al., 1998; Urashima et al., 1997), 6’-SLN was isolated from caprine colostrum (Urashima et al., 1997) and 6’-Neu5GCLN was isolated from addax colostrum (Ganzorig et al., 2018).
To harvest carbohydrate fraction from colostrum samples, colostrum samples were thawed and dissolved with four volumes of chloroform/methanol (2:1, v/v). The mixture was then centrifuged at 4°C and 4,000×g for 30 min. Only the dissolved component in methanol was collected. Methanol was removed from the component by rotary evaporation, and the residue was dissolved in 5 mL of ion exchange water. The residue was then freeze dried. The lyophilized white powder designated as the carbohydrate fraction. The carbohydrate fractions from dairy goat colostrum from Farms I and II were then named as EGCI and EGCII, respectively.
The lyophilized carbohydrate fraction of each sample was then dissolved by using 2 mL of ion exchange water. The solution of carbohydrate fraction of each sample was filtered and then applied to gel filtration by using BioGel P-2 (<45 μm, Bio Rad Laboratories, Hercules, CA, USA) column (φ 2.5×100 cm) at a flow rate of 0.25 mL/m. Ion exchange water was used as mobile phase. Fraction collector with 95 installed test tubes was used to collect 5 mL of fraction in every 20 minutes. The aliquots of each fraction were then subjected to phenol-sulfuric acid test (DuBois et al., 1956) at 490 nm for hexose analysis and for sialic acid analysis by the periodate-resorcinol test (Jourdian et al., 1971) at 630 nm. All peak fractions generated from both tests, were pooled and lyophilized. Fig. 1 depicted the carbohydrate fraction profile extracted from EGCI and EGCII samples.
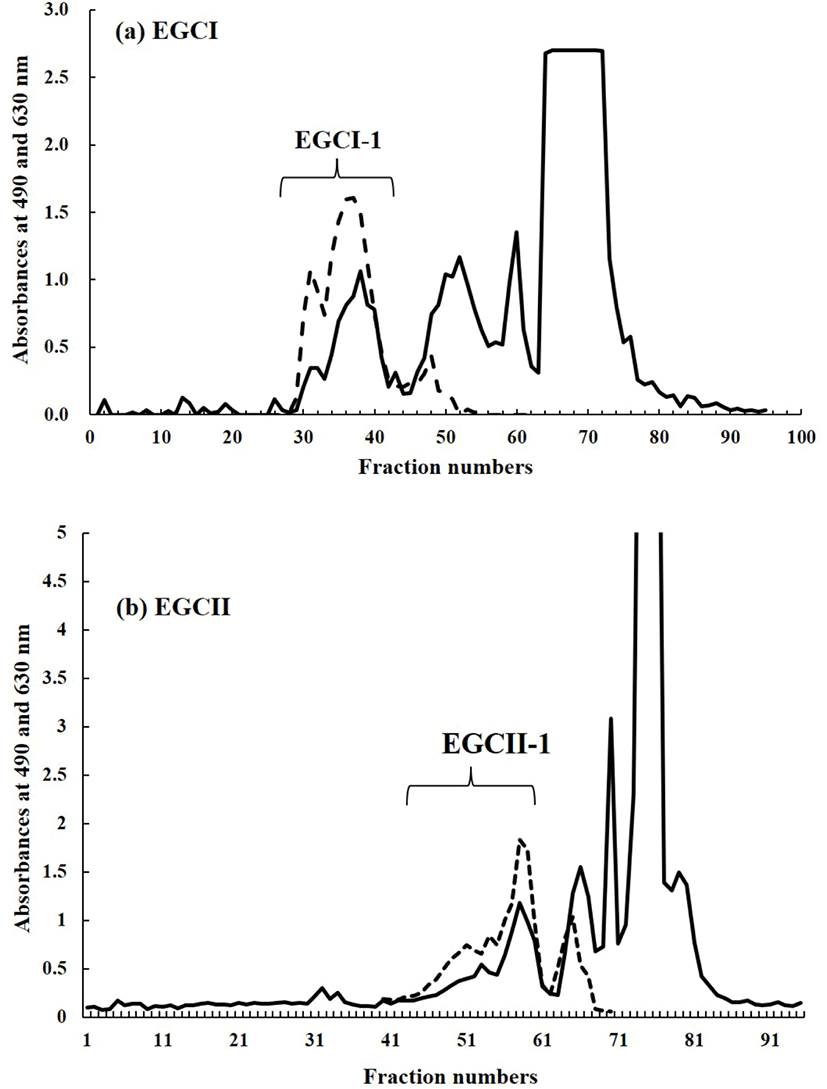
The components which reacted positively to the periodate-resorcinol method for testing sialic acid (Fig. 1), were separated by high-performance liquid chromatography (HPLC) on a TSK Amide-80HR gel column (4.6×250 mm, pore size 80Å, particle size 5 μm: Tosoh, Tokyo, Japan) using an L-2130 pump (Hitachi, Tokyo, Japan). The HPLC for both components from EGCI and EGCII were performed using mobile phase 80% and 50% (v/v) acetonitrile in 15 mmol/L potassium phosphate buffer (pH 5.2) labelled as buffer A and buffer B, respectively. Elution was done using a linear gradient of acetonitrile from buffer A to buffer B, 80%−50% at 60°C at a flow rate of 1 mL/min. UV detector was used to monitor the eluates by measuring the absorbance at 195 nm. The peak fractions of oligosaccharides (Fig. 2) were collected, concentrated by rotary evaporation, and then subjected to proton or 1H-NMR for analysis to determine their chemical structures.
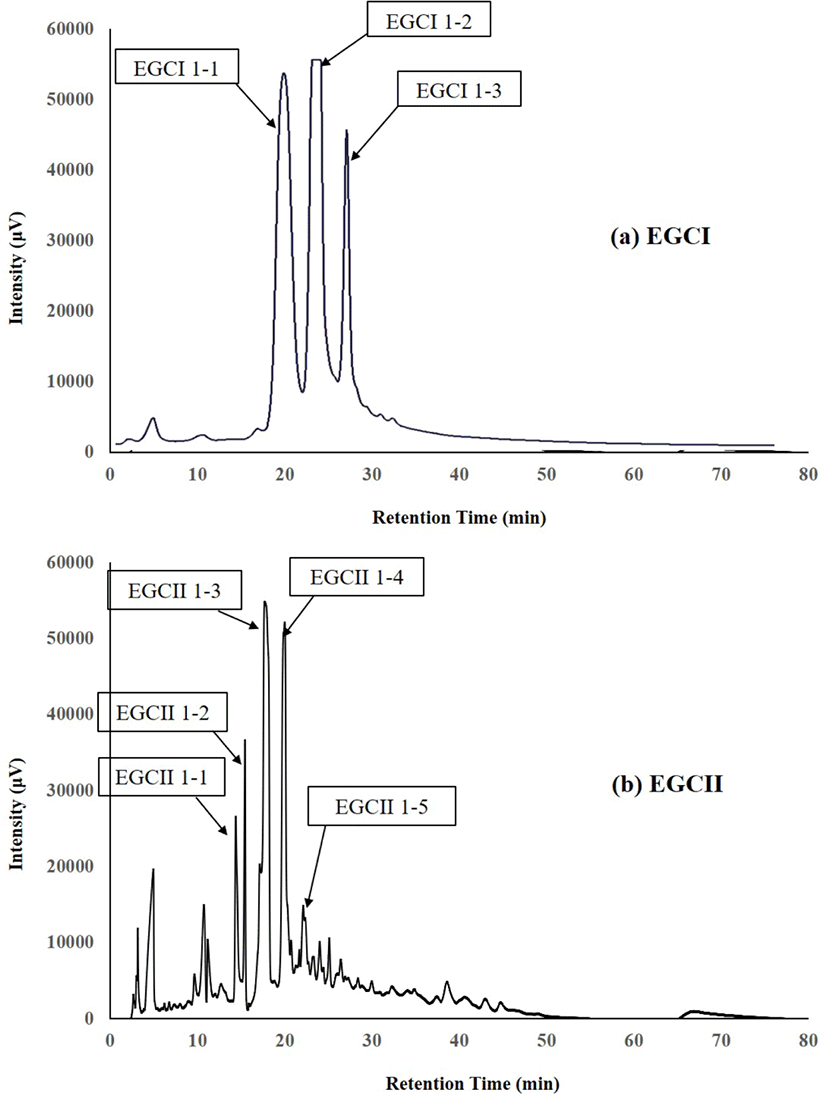
All spectra of nuclear magnetic resonance (NMR) were recorded in D2O (99.96 atom D%; Sigma-Aldrich, Milwaukee, WI, USA) at 600 Mhz for proton/1H-NMR with a Varian INOVA 600 spectrometer (Varian, Palo Alto, CA, USA) operated at 293.1 K. Chemical shifts are expressed at the change relative to internal 3-(trimethyl)-1-propane sulfuric acid, sodium salt, but measured by reference to internal acetone (δ=2.225).
Results
Fig. 1 shows the chromatogram of the carbohydrate fractions extracted from EGC of EGCI and EGCII as result of gel filtration by using BioGel P-2. The fraction from each sample was separated into four peaks. However, as the focus of the present study is to characterize SOS, only the first peak or fraction from each sample pooled and labelled as EGCI-1 and EGCII-1, respectively (Fig. 1). Those two fractions were assumed to contain SOS due to their positive reaction with the periodate-resorcinol test.
The components in EGCI-1 and EGCII-1 from EGC separated into several peaks by normal-phase HPLC as shown in Fig. 2. The peaks separated from EGCI-1 were designated as EGCI 1-1, EGCI 1-2 and EGCI 1-3, whereas peaks separated from EGCII-1 were designated as EGCII 1-1, EGCII 1-2, EGCII 1-3, EGCII 1-4, and EGCII 1-5.
The proton NMR spectrum [chemical shift (ppm) and coupling constant (Hz) in Table 2] of this oligosaccharide in EGCI 1-1 had anomeric shifts at δ 5.222, 4.663, and 4.530 which were assigned to αGluc, βGluc, and β1-4-linked Gal, while the shifts at 1.800, 2.756, and 2.030 were assigned to H-3 axial and equatorial, and NAc shifts of α2-3-linked Neu5Ac. As these shifts were identical to that of original 3’-Neu5AcLac or 3’-SL, this fraction was therefore characterized to be Neu5Ac(α2-3)Gal(β1-4)Glc.
The spectrum [chemical shift (ppm) and coupling constant (Hz) in Table 2] of this oligosaccharide in EGCI 1-2 had shifts at δ 5.225, 4.669, and 4.427 arising from the H-1 of αGluc, βGluc, and β1-4-linked Gal, respectively. The spectrum’s major shifts were H-3 axial and equatorial, and NAc shifts at δ 1.743, 2.711, and 2.028, respectively. As these shifts were similar to those of authentic 6’-Neu5AcLac or 6’-SL, the oligosaccharide in this fraction was characterized as Neu5Ac(α2-6)Gal(β1-4)Glc.
The spectrum [chemical shift (ppm) and coupling constant (Hz) in Table 2] of this oligosaccharide in EGCI 1-3 had H-3 axial and equatorial shifts at δ 1.762 and 2.731, respectively, of a sialic residue, indicating it contains an α2-6 sialyl unit. The spectrum does not contain the NAc shif at δ 2.031 but has a shift at δ 4.118, which is deduced to be due to an NGc group of sialic acid. The shifts at δ 5.227, 4.671 and 4.431 were assigned to the H-1 of αGluc, βGluc, and β1-4-linked Gal, respectively. These shifts are similar to those of the GS-3 fraction from Japanese Saanen colostrum isolated by Urashima et al. (1997), and was therefore characterized as Neu5Gc(α2-6)Gal(β1-4)Glc.
The 1H-NMR spectrum [chemical shift (ppm) and coupling constant (Hz) in Table 3] of this oligosaccharide in EGCII 1-1 had the characteristic doublet-doublet shift at δ 5.424 and a singlet peak at δ 2.052. As this NMR spectrum pattern was similar to those of authentic GlcNAc-1-P, it was thought that this fraction contained GlcNAc-1-P. However, it was shown that the fraction contained another component, because the spectrum had chemical shift at δ 5.223, 4.666, and 4.452, which arose from the αGluc, βGluc, and β1-4-linked Gal of another saccharide. Therefore, this fraction could not be characterized at this stage.
The 1H-NMR spectrum [chemical shift (ppm) and coupling constant (Hz) in Table 3] had anomeric shifts of αGluc, βGluc, and β1-4-linked Gal at δ 5.223, 4.666, and 4.451, respectively. The chemical shifts of H-3 axial and equatorial, and NAc of α2-6-linked Neu5Ac were detected at δ 1.722, 2.669, and 2.027, respectively. However, the saccharide in this fraction was not characterized at this staged, because these shifts of axial and equatorial H-3 anomer were differed from chemical shifts of Neu5Ac(α2-6)Gal(β1-4)Glc (6’-Neu5AcLac) at δ 1.745 and 2.712.
Based on the 1H-NMR spectrum signals (Fig. 3), it was concluded that the fraction contained two oligosaccharides. Table 3 shows the assignment of the chemical shifts of the components in peaks for EGCII 1-3 and 1-4. The anomeric shifts at δ 5.221, 4.663, and 4.531 were assigned to αGluc, βGluc, and β1-4-linked Gal, while the shifts at δ 1.799, 2.755, and 2.027 were assigned to H-3 axial and equatorial, and NAc shifts of α2-3-linked Neu5Ac. These shifts were identical to those of original 3’-Neu5AcLac or 3’-SL, this fraction is therefore characterized as Neu5Ac(α2-3)Gal(β1-4)Glc (EGCII 1-3-1). Other anomeric shifts were identified in this spectrum at δ 5.196, 4.749, and 4.551 of αGlcNAc, βGlcNAc, and β1-4-linked Gal, respectively, with H-3 axial and equatorial shifts at δ 1.719 and 2.667, respectively. As the shifts were similar to those of the major anomeric shifts for 6’-Neu5AcLacNAc or 6’-SLN of the GS-1 fraction from Japanese Saanen colostrum isolated by Urashima et al. (1997), this component was characterized as Neu5Ac(α2-6)Gal(β1-4)GlcNAc (EGCII 1-3-2). The spectrum also had an NAc shift of GlcNac at δ 2.063. It was concluded that the amounts of 3’Neu5AcLac and 6’-Neu5AcLacNAc in this fraction were rather similar, as the areas of H-3 equatorial shift of α2-3-linked Neu5Ac and α2-6-linked Neu5Ac were similar to each other.
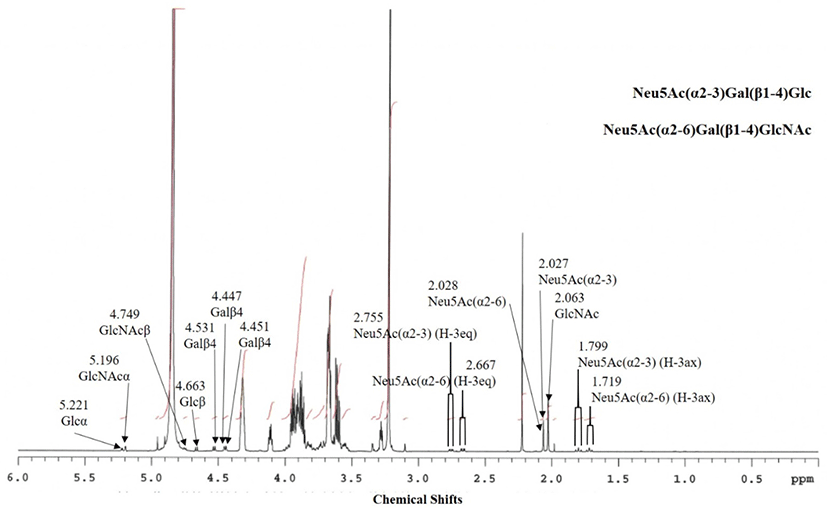
Based on the 1H-NMR spectrum [chemical shift (ppm) and coupling constant (Hz) in Table 3], this fraction contained a major component as well as minor component. The anomeric shifts at δ 5.224, 4.669, and 4.427 arose from H-1 of αGluc, βGluc, and β1-4-linked Gal of the major component in the fraction. The spectrum had major shifts of H-3 axial and equatorial and NAc shifts at δ 1.745, 2.712, and 2.030, respectively. Due to similarity of chemical shifts of 6’-Neu5AcLac of the previously isolated GG-1-1-2 fraction from Greater Galago milk (Taufik et al., 2012), the major component of this fraction was characterized to be Neu5Ac(α2-6)Gal(β1-4)Glc (EGCII 1-4-1). The spectrum also had anomeric shifts, which arose from a minor saccharide, of βGluc, and β1-4-linked Gal at δ 4.664 and 4.535, respectively. Chemical shifts of H-3 axial and equatorial and NGc of α2-3-linked Neu5Gc were also identified in this spectrum at δ 1.817, 2.774, and 4.115, respectively. Based on these shifts, the 2nd oligosaccharide was characterized to be Neu5Gc(α2-3)Gal(β1-4)Glc (3’-Neu5GcL, EGCII 1-4-2). In addition, other anomeric shifts had been identified at δ 5.119, 4.446, and 4.452 of αGlcNAc and β1-4-linked Gal with H-3 axial and equatorial and NGc shifts at δ 1.745, 2.686, and 4.119, respectively. Based on these chemical shifts, the third saccharide in this fraction was characterized as Neu5Gc(α2-6)Gal(β1-4)GlcNAc (6’-Neu5GcLN, EGCII 1-4-3). The anomeric shifts of this fraction were similar to those from the AC 2-5-3 fraction of addax colostrum reported by Ganzorig et al. (2018).
Discussion
The major SOS characterized in this EGC were 3’-SL, 6’-SL, 6’-Neu5GcL, and 6’-SLN, while the minors were 3’-Neu5GCL and 6’-Neu5GcLN. Compared with the MOS in bovine colostrum, the ratio of 6’-SLN to 3’-SL in this caprine colostrum was rather high, because there is more 3’-SL than 6’-SLN in bovine colostrum (Nakamura et al., 2003). These findings are in line with the report on gMOS composition by Sousa et al. (2019b).
One of the major SOS in bovine colostrum, namely Neu5Acα2-8Neu5Acα2-3Galβ1-4Glc, was not found in this study. However, it is assumed that this saccharide might be contained in this colostrum as a minor component. If one used a mass spectrum-based technique for structure analysis, this might be detected, too.
All the gMOS characterized in this study have been found in caprine milk and colostrum in previous studies (Albrecht et al., 2014; Chatziioannou et al., 2021; Lu et al., 2020; Urashima et al., 1994; Urashima et al., 1997; van Leeuwen et al., 2020). This is to confirm that different proportions of SOS (Claps et al., 2014; Claps et al., 2016), share a common profile of neutral and acidic/SOS as they belong to the same species of Capra hircus.
Many of the previous studies showed that there are some common structures between gMOS and hMOS. Four out of then neutral (β3’-galactosyllactose, β6’-galactosyllactose, 2’-fucosyllactose, Lacto-N-hexaose) and two out of seven acidic (3’-N-acetylneuraminyllactose/3’-SL and 6’-N-acetylneuraminyllactose/6’-SL) were found in milk of both species. Moreover, the Lacto-N-Biose (LNB) unit—the building block of type I hMOS—has also been identified in gMOS (Kiskini and Difilippo, 2013; Sousa et al., 2019b).
gMOS have a more similar profile to those of hMOS as compared to those in bMOS and sMOS. Therefore, gMOS was considered to be the best natural source of oligosaccharides for infants (Leong et al., 2019; Silanikove et al., 2010; van Leeuwen et al., 2020). Some studies have been proved that oligosaccharides containing sialic acid residues or SOS acted as receptor analogue, playing an important role in brain development, and increasing immunity in infants (Bode et al., 2004; Boehm and Stahl, 2007; Yue et al., 2020).
Another characteristic of gMOS that has been reported by some studies showed that fucosylated and sialylated oligosaccharides were available in higher concentration in goat milk or colostrum than in those from other ruminants (Martinez-Ferez et al., 2006a; Martinez-Ferez et al., 2006b; Meyrand et al., 2013). Up to date, the number of identified oligosaccharides structure from goat was 40, and the quantity was 200 to 650 mg/L in colostrum, and 60 to 350 mg/L in mature milk (van Leeuwen et al., 2020). These levels of gMOS’s quantity were significantly lower than those of hMOS (5−20 g/L) but approximately five times greater than bMOS and about 10 times greater than sMOS (Albrecht et al., 2014; van Leeuwen et al., 2020).
SOS concentration ranges from 1 g/L in colostrum to 90–450 mg/L in human mature milk (Martín-Sosa et al., 2003). In goat mature milk, it ranges from 120 to 190 mg/L (Martinez-Ferez et al., 2006b) and in colostrum from 144 to 328 mg/L (Claps et al., 2014; Claps et al., 2016). Among SOS, two of the most representative in human milk/colostrum, similarly in goat milk/colostrum, were 3’-SL and 6’-SL. The concentration of 3’-SL and 6’-SL in hMOS is 100−200 and 1,140−1,310 mg/L, respectively, whereas in gMOS it was 30−50 and 50−70 mg/L, respectively (Kiskini and Difilippo, 2013).
Recently, Huang et al. (2021) reported that 3’-SL and 6’-SL extracted from hMOS showed the ability to significantly reduce apoptosis, restore proliferation, and relieve inflammation of the intestinal cells of neonatal mice under hypoxia. They also indicated that 3’-SL and 6’-SL provided protection against NEC-induced intestinal damage by deactivation of the TLR4/NF-κB pathway. In this study with EGC, we successfully characterized 3’-SL from fractions EGCI 1-1 and EGCII 1-3-1 and 6’-SL from fractions EGCI 1-2 and EGCII 1-4-1 (Table 1) as two major SOS. Based on the above reports, 3’-SL and 6’-SL isolated from goat milk colostrum are suggested to have similar bioactivity to those isolated from human milk or colostrum as they had exactly similar chemical structures.
Several studies on goat milk-based infant formula in comparison to cow milk-based infant formula have been done to investigate the bioactivity, such as prebiotic and anti-infection properties. Among them are two recent studies reported by Leong et al. (2019) and Urakami et al. (2018). Urakami et al. (2018) assessed the sialyl and neutral oligosaccharides from goat and bovine milk and colostrum for use as ingredients for infants. They concluded that gMOS have more potential as ingredients of infant formula or functional foods due to their potency of adherence inhibition, safety, and the availability. Leong et al. (2019) reported that MOS naturally available in goat milk-based infant formula showed strong prebiotic and anti-pathogen adhesion capability which may deliver gut health benefit to infants.
Conclusion
All of the previously mentioned research data showed that goat milk and colostrum are naturally available sources of human-like oligosaccharides. It is both the structural profile and higher content of MOS in milk and colostrum from goats, as opposed to other domestic dairy animals, that places gMOS in the first ranks of animal-derived oligosaccharides. However, data concerning the variation of gMOS profile depending on diet, lactation stage, season, breed, and number of milking still need to be collected. Potential variations in gMOS composition and concentration could be extremely important for their future use. In this study, we successfully characterized the following oligosaccharides from EGC: Neu5Ac(α2-3)Gal(β1-4)Glc (3’-N-acetylneuraminyllactose), Neu5Ac(α2-6)Gal(β1-4)Glc (6’-N-acetylneuraminyllactose), Neu5Gc(α2-3)Gal(β1-4)Glc (3’-N-glycolylneuraminyllactose), Neu5Gc(α2-6)Gal(β1-4)Glc (6’-N-glycolylneuraminyllactose), Neu5Ac(α2-6)Gal (β1-4)GlcNAc (6’-N-acetylneuraminyllactosamine), and Neu5Gc(α2-6)Gal(β1-4)GlcNAc (6’-N-glycolylneuraminyllactosamine). Therefore, EGC and milk are among the readily available natural sources in Indonesia for extracting bioactive MOS to be used in the food or pharmaceutical industries. Further study to investigate and confirm the array and quantity of neutral and acidic oligosaccharides from colostrum and milk of Etawah Grade, as well as other local dairy goat breeds in Indonesia, is of central importance.