Introduction
Nowadays, consumers are becoming interested in food products containing probiotics, which provide a health benefit. Yogurt and fermented milk have been known as probiotic dairy foods that can enhance digestion, boost immunity, and provide other health benefits (Burgain et al., 2013; Figueroa‐González et al., 2011). Global functional food market is estimated to have an increase up to 253 billion USD by 2024 as it is consistently growing at a pace of 5% to 20% depending on the type of products (Dixit et al., 2016; Lachowicz et al., 2020). Probiotics are defined as “live microorganisms which confers a health benefit on the host when consumed in adequate amounts” and have been widely applied to various dairy foods including yogurt, cheese and ice cream and non-dairy products including chocolate and juices (Abbaszadeh et al., 2014; Burgain et al., 2011). For dairy food application, probiotics are commonly freeze-dried for long-term storage and cryoprotectants were added to probiotic suspensions to minimize freeze damage (Fenster et al., 2019). Skim milk powder, a source of milk protein, have been widely used as a common cryoprotectant for probiotics in dairy industry (Fenster et al., 2019).
Several studies have shown that Lactobacillus rhamnosus GG (LGG) has excellent intestinal mucus adherence capacities, prevention, and treatment of gastrointestinal infections and diarrhea (Chávarri et al., 2010; Segers and Lebeer, 2014). However, to provide health benefits to the host, a sufficient number of viable bacteria are needed to survive until reaching the upper gastrointestinal tract (Librán et al., 2017). Since harsh conditions during food processing including pasteurization, storage, and digestion can reduce the viability of probiotics (Ha et al., 2016; Heidebach et al., 2009), it is critical to maintain the probiotic viability until the end of shelf life and reaching intestinal tract (Mattila-Sandholm et al., 2002; Ross et al., 2005). In particular, pasteurization has been used to remove the risk of pathogens in food processing. Therefore, it is important to provide effective protection against this heat treatment to probiotics (Su et al., 2021).
Various probiotic delivery systems have been developed to protect and enhance the viability of probiotics using encapsulation techniques. The one of the most extensively used encapsulation techniques for probiotics is high-temperature spray drying (Burgain et al., 2015). However, the use of high-temperature treatment (>130°C) during spray-drying can decrease the viability of probiotics (Ross et al., 2005) and produce relatively lager size (e.g., 200–1,000 μm) of probiotic delivery system that can negatively affect the sensorial acceptability of applied food products (Anal and Singh, 2007; Krasaekoopt et al., 2003). Therefore, it is beneficial to develop probiotic delivery systems using low-temperature treatment (e.g., <~40°C). In this study, milk protein-based delivery system (MPDS) was manufactured by using chymosin-induced gelation with a safe cross-linking agent (CaCl2). Since chymosin-induced gelation method uses relatively low temperature heat treatment (e.g., below 40°C), it is beneficial to encapsulate heat-sensitive substances including probiotics for food applications (Burgain et al., 2011; Esteves et al., 2003; Gardiner et al., 1998; Stanton et al., 1998).
We hypothesized that reaction temperature and holding time, which can affect the chymosin activity and milk protein association, may play an important role in determining the physicochemical and functional properties of MPDS containing LGG, during manufacturing and pasteurization process. The aims of this research were to manufacture probiotic delivery system, MPDS, using chymosin-induced gelation and to study how manufacturing variables, such as reaction temperature and holding time, affected the physicochemical properties and viability of LGG encapsulated in MPDS during manufacture, pasteurization process, and in vitro digestion.
Materials and Methods
Skim milk powder was kindly donated from Seoul Milk Cooperative (Seoul, Korea). Chymosin was obtained from Natural standard plus 290, Hansen Pty (Blenheim, New Zealand), CaCl2, and Span 80 were purchased from Sigma-Aldrich (St. Louis, MO, USA).
All glass wares used in microbial culture were sterilized at 121°C for 15 min. LGG was cultured in de Man, Rogosa, and Sharpe (MRS) broth media (Difco Laboratories, Sparks, NV, USA) at 37°C for 18 h. After two subcultures in MRS broth media, the cell suspension was centrifuged at 1,500×g, 4°C for 5 min. The pellet was washed twice with sterile 0.9% (w/v) sodium chloride solution and then used for the further encapsulation process.
MPDS containing LGG was produced using chymosin-induced gelation method modified and described in previous study (Heidebach et al., 2009). MPDS was manufactured using 5% (w/w) of skim milk solution that reconstituted in distilled water. Skim milk solution was adjusted to pH 5.4 using 1 M HCl and cooled to 5°C for 1 h. Collected LGG was suspended in skim milk solution to obtain LGG/skim milk mixture with an initial amount of at least 9.0 Log CFU/mL of LGG. Next 51.7 μL of chymosin was added to 15 mL of LGG/skim milk mixtures and then kept at 5°C for 1 h. Seventy-five microliters of 1 M CaCl2 was added to 160 g of soybean oil containing 5% (w/w) span 80 and homogenized at 8,000 rpm for 5 min. The temperature was kept at 5°C during homogenization to prevent further chymosin-induced gelation. After the formation of water in oil (W/O) emulsions, the temperature of emulsion was adjusted to 25°C, 30°C, 35°C, and 40°C and then kept for 5, 10, 20, and 30 min at 25°C to induce gelation of the milk protein by chymosin. To obtain MPDS, W/O emulsions were centrifuged at 15,000×g, 4°C for 1 min and oil at the top layer was removed. After washing three times with distilled water, MPDS containing LGG was collected and stored at –80°C before freeze-dried.
Confocal laser scanning microscope (CLSM, Olympus FV-1000, Tokyo, Japan) was used to determine the morphological properties of MPDS. Acridine orange was used as a fluorescent milk protein dye. Ninety microliters of 0.2% (w/w) acridine orange were added to 15 mL of skim milk solution treated with various reaction temperatures and holding times. The excitation and emission wavelengths were 488 and 526 nm, respectively.
Particle size analyzer (1090LD shape, CILAS, Paris, France) was used to measure the particle size (volume-mean diameter, d43) and span value (size distribution) of MPDS. The span value of MPDS was obtained from D90, D10, and D50 value, which are volume size diameters at 90%, 10%, and 50% of the cumulative volume, respectively. Span value was determined as expressed in equation (1) (Rastinfard et al., 2018).
The encapsulation efficiency (EE) of LGG in MPDS was evaluated by counting the number of viable cells using a standard plate culture method on MRS agar at 37°C for 48 h. EE of LGG in MPDS was determined by the following equation (2) (Chávarri et al., 2010).
Where N0 is the initial amount of LGG added in the preparation process and N is the total amount of LGG in MPDS enumerated as Log CFU/mL. The initial amount of LGG was obtained according to the microbial culture process.
To measure the viability of LGG in MPDS during pasteurization, 0.1 g of free and encapsulated LGG in MPDS were mixed into 10 mL of 5% (w/w) skim milk solution and then were heated at 65°C for 30 min. The viable cells of LGG before and after pasteurization were counted using standard plate method on MRS agar.
The viability of LGG during in vitro digestion was assessed using the modified method of Chávarri et al. (2010). Simulated gastric juice (SGJ) was prepared with 9.0 g/L of sodium chloride containing 3.0 g/L of pepsin and then adjusted to pH 2.0. Simulated intestinal juice (SIJ) was composed of 3.0 g/L bile salts, 6.5 g/L NaCl, 0.835 g/L KCl, 0.22 g/L CaCl2 and 1.39 g/L NaHCO3 at pH 7.5. Free LGG and LGG encapsulated in MPDS were diluted 10-fold with SGJ and SIJ and then incubated at 37°C for 120 min with constant stirring at 150 rpm. The number of viable cells was counted using a standard plate culture method on MRS agar at 37°C for 48 h.
Results are presented as mean±SD of three replicates. One-way analysis of variance (ANOVA) with Fisher’s Least Significant Differences (LSD) test was used to determine the effects of reaction temperature and holding time on the particle size and span value of MPDS, EE, and viability of encapsulated LGG during pasteurization and in vitro digestion. Statistical significance was set at 5% level (p<0.05). All analyses were performed using the SPSS software package (SPSS 20.0 for Windows; SPSS, Chicago, IL, USA).
Results and Discussion
Chymosin, a proteolytic enzyme, can hydrolyze negatively charged κ-casein existing at the surface of casein micelles. It leads to the reduction of electrostatic repulsion between casein micelles and enhances intermolecular associations between casein micelles forming chymosin-induced gel. In this study, milk protein solutions were adjusted to pH 5.4, where near the optimum pH 5.8 for chymosin (Fox, 1969). In CLSM images, MPDS had mostly round shape with rough surface and contained a diameter ranging from ~7 to 28 μm indicating that MPDS was successfully manufactured (Fig. 1).
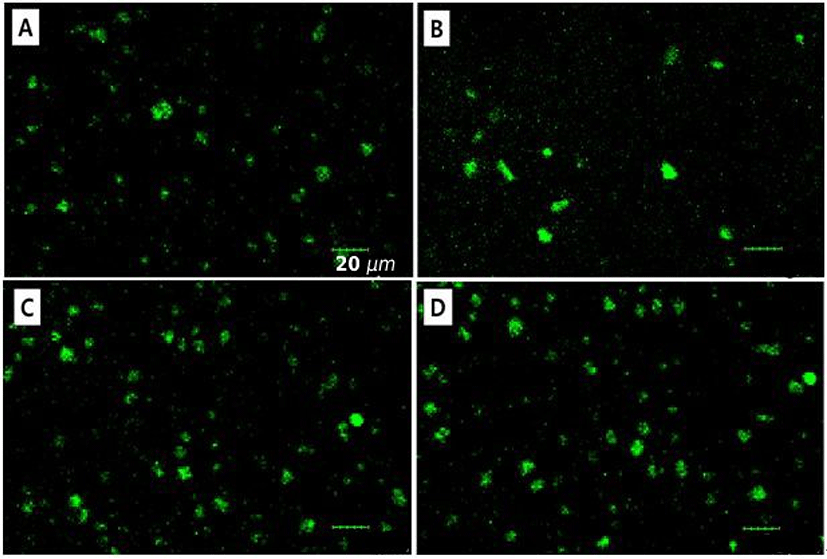
The impacts of reaction temperature and holding time on the physicochemical properties of MPDS, such as particle size and span value, were shown in Figs. 2 and 3. Particle size and span value (size distribution) are crucial factors to affect the functional properties of delivery systems, such as their physical stability, sensory attrribute in foods, bioavailability of encapsulated compounds (Anal and Singh, 2007; Krasaekoopt et al., 2003). For example, it was expected that the reduction of particle size of MPDS could enhance the physical stability during manufacturing and storage.
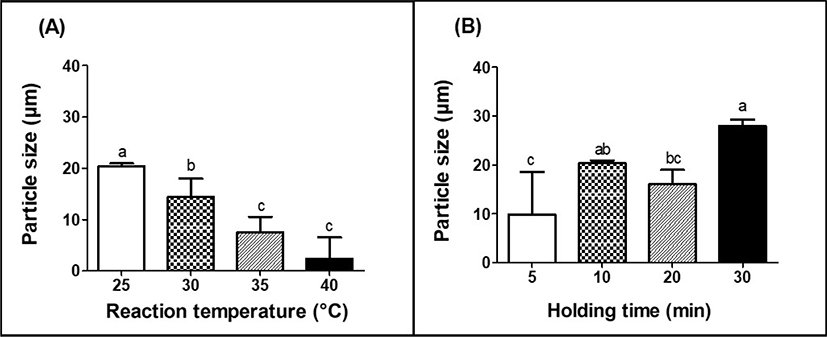
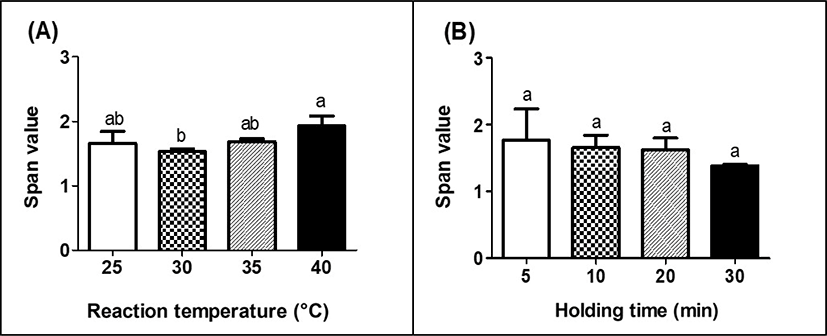
The particle size of MPDS was significantly (p<0.05) decreased from 20.4 to 5.4 μm as the reaction temperature was increased from 25°C to 40°C (Fig. 2A). Hydrophobic associations between casein micelles are major forces for their aggregations and are strongly temperature dependent (Fox, 1969). An increase in reaction temperature from 25°C to 40°C could enhance the hydrophobic associations between casein micelles (Bansal et al., 2007; Lucey, 2011), which may contribute to the shrinkage of casein-based complexes and formation of smaller MPDS.
In other hands, the particle size of MPDS was significantly (p<0.05) increased from 9.9 to 28 μm with an increase in holding time from 5 to 30 min at 25°C (Fig. 2B). In this study, after the loss of the hydrophilic domain from κ-casein, individual casein micelles flocculate leading to the development of casein aggregates. An increase in the hydrolysis of κ-casein with an increase in holding time may result in an increase in the aggregations of casein micelles during chymosin-induced coagulation and the formation of much bigger particles.
Fig. 3 presents the effects of reaction temperature and holding time on the span value of MPHS. Span value in Fig. 3 is an indicator to provide the basis to assess the homogeneity and size distribution of particles [26]. Low span value indicates a narrow and homogeneous size distribution (Chew and Chan, 2002).
There was no significant (p<0.05) difference on span value with an increase in reaction temperature although the span value of MPDS treated at 40°C was higher than that of MPDS treated at 30°C (Fig. 3A). No significant (p>0.05) differences on span value were observed as holding time was increased from 5 to 30 min (Fig. 3B). All MPDS had span value ranging from 1.4 to 2.1, which indiates that MPDS had a narrow and homogeneous size distribution (Gallotti et al., 2020).
The EE of LGG in MPDS was shown in Fig. 4. The EE of LGG in MPDS was significantly (p<0.05) increased from 66.6% to 80.5% as the reaction temperature of the MPDS was increased from 25°C to 40°C (Fig. 4A). An increase in hydrophobic associations between milk proteins at higher reaction temperature may lead to the formation of denser and more stiff protein gel structures. It can reduce the diffusion of LGG out of MPDS and enhance the EE of LGG in MPDS, which results in increased EE of LGG. The EE of LGG was significantly (p<0.05) increased from 67.1% to 75.2%, as the holding time of the MPDS was increased from 5 to 30 min (Fig. 4B). The EE of LGG can be affected by various factors, such as particle size, concentration of capsule making solution, probiotics cell load, and hardening time (time needed for capsule formation) (Chávarri et al., 2010; Solanki et al., 2013). In this study, increased the EE of LGG in MPDS was accomplished due to the excellent gelation properties of milk protein during enzymatic process with chymosin. Longer holding time during gelation process can lead to an increase in κ-casein hydrolysis and aggregations of casein micelles, which may enhance the density of the gel network. Since the formation of denser protein gel structures treated with longer holding time could protect and encapsulate more LGG inside of MPDS, the higher EE of LGG in MPDS was obtained at longer holding time (Heidehach et al., 2012).
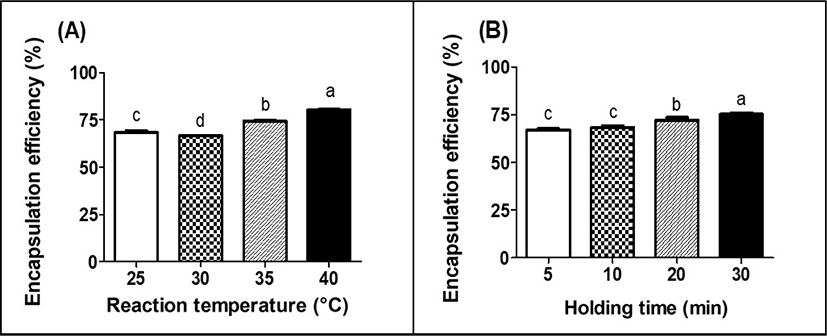
Comparing the EE of LGG in MPDS with data from other literatures, it can be stated that the EE of LGG in MPDS was relatively higher. The EE of LGG in chitosan-coated alginate microcapsule produced with extrusion technique was about 25%–53% (Abbaszadeh et al., 2014). In other hands, the EE of bifidobacteria in whey protein-based microcapsules was 0.71%–25.7% after spray-drying. The low EE of bifidobacteria could be due to high temperature (± 160°C) during encapsulation using spray drying process (Picot and Lacroix, 2004). Compared with the EE of probiotics in microcapsule produced with extrusion or spray drying process, higher the EE of probiotics in MPDS could be due to lower reaction temperature.
For food applications, probiotics are usually incorporated into dairy products, such as fermented dairy beverage and yogurt. Pasteurization has been extensively used to eliminate pathogenic microorganisms and extend the shelf life of various types of foods. However, pasteurization can negatively affect the viability of probiotics (Su et al., 2021). Therefore, it is important to ensure that the viability of encapsulated probiotics is to be maintained in food during pasteurization (Teoh et al., 2011). The viability of encapsulated LGG and LGG without MPDS (free cell) in skim milk after pasteurization at 65°C for 30 min was presented in Fig. 5. The viability of LGG without MPDS was significantly (p<0.05) decreased to 63.9% after pasteurization. On the other hand, LGG encapsulated in MPDS had significantly (p<0.05) higher viability than LGG without MPDS (Fig. 5). An increase in reaction temperature and holding time resulted in a significant (p<0.05) increase in the viability of LGG encapsulated in MPDS after pasteurization (Fig. 5).
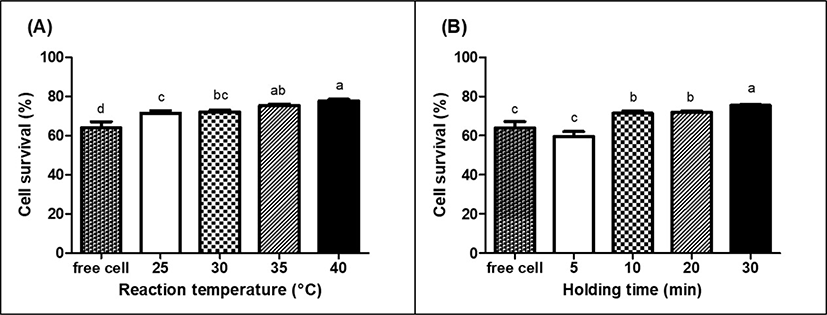
Higher LGG viability encapsulated in MPDS would be due to the protective effect of chymosin-induced protein gel networks for LGG, which could enhance the thermal resistance of LGG. Similar result was reported that the viability of encapsulated probiotics, Lactobacillus acidophilus LA-5 and Bifidobacterium pseudocatenulatum G4, in alginate microcapsule was significantly (p<0.05) higher (≥78%) than that of probiotics without alginate microcapsule during pasteurization at 65°C for 30 min (p<0.05), suggesting that encapsulation using alginate was a feasible method for the effective protection of probiotics against pasteurization (Teoh et al., 2011).
Probiotics will undergo a complex series of physical and chemical changes while they go through the gastrointestinal tracts after ingestion. The maintenance of probiotics viability throughout the gastrointestinal tract is one of the major issues in food industry (Burgain et al., 2015). In this study, the viability of free and encapsulated LGG in MPDS during in vitro digestion were evaluated in simulated gastric- and intestinal juice (Figs. 6 and 7). During incubation in SGJ for 120 min, the viability of free LGG was gradually decreased from 8.96 to 5.61 Log CFU/mL indicating that the highly acidic condition of stomach negatively affected the viability of LGG. In Fig. 6, the encapsulation of LGG in MPDS resulted in a significant increase in the viability of LGG during incubation in SGJ. It was found that more LGG were survived when they were encapsulated in MPDS manufactured with higher reaction temperature (Fig. 6A) and longer holding time (Fig. 6B). As we described earlier, an increase in reaction temperature and holding time could lead to the formation of denser protein gel network, which could protect LGG more efficiently against the highly acidic condition of SGJ (Heidehach et al., 2012).
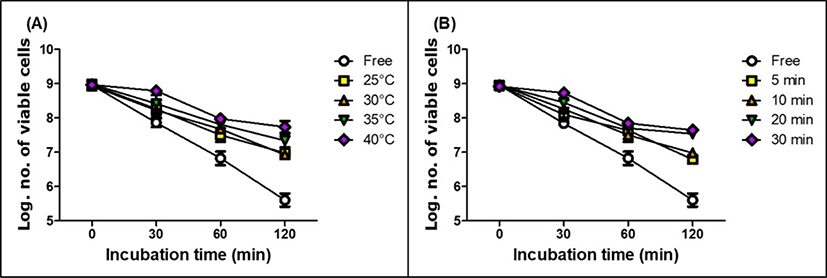
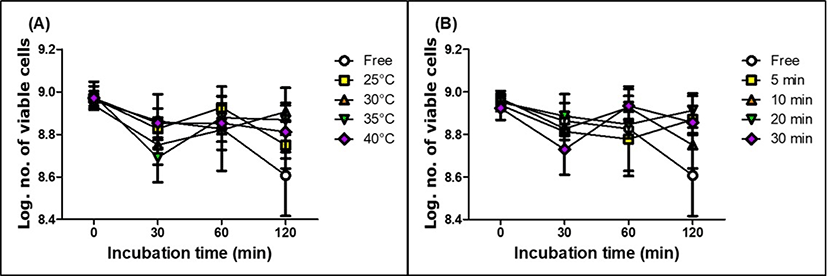
The viability of free and encapsulated LGG in MPDS during incubation in SIJ was shown in Fig. 7. There were no significant effects on the viability of free and encapsulated LGG in MPDS indicating that LGG could survive well in intestinal condition.
Conclusion
It can be concluded that MPDS was successfully manufactured using chymosin-induced gelation at various reaction temperatures from 25°C to 40°C and holding times from 5 to 30 min. Reaction temperature and holding time were the key parameters that affected the morphological and physicochemical properties, such as particle size and span value, of MPDS. It was found that the EE of LGG in MPDS was enhanced as reaction temperature and holding time were increased. The use of MPDS can protect and maintain the viability of LGG during pasteurization and in vitro digestion under stomach condition. Overall, it was valuable to develope probiotic delivery sytem, MPDS, using chymosin-induced gelation method, which is low temperature treatment for food application. Further studues are needed to investigate the effect of MPDS encapsulation on the viability of probiotics during storage in various foods.