Introduction
Freezing is often performed to enable storage of aquatic products. Freezing extends the shelf life of aquatic products (Zuanazzi et al., 2019), and also reduces the degree of lipid degradation and oxidation (Ali et al., 2015). However, during transportation and sales, products may experience cycles of freezing and thawing, potentially leading to cell damage (Benjakul and Bauer, 2001) and seriously affecting fish quality (Jiang et al., 2019; Wangtueai and Maneerote, 2018).
Surimi products are a popular kind of aquatic products. During the processing of surimi products, pork back fat is often added to improve the overall sensory quality (Chojnicka et al., 2009). However, this added fat may also promote lipid oxidation, thus affecting the food quality and nutritional value of the product (Pietrowski et al., 2011; Shi et al., 2014). During lipid oxidation, free radicals are produced. Jiang et al. (2020) found that free radicals were formed first during lipid oxidation, followed by primary oxidation products such as hydroperoxides and secondary oxidation products such as thiobarbituric acid reactive substances (TBARS). However, to the best of our knowledge the relationship between lipid degradation and lipid oxidation has not been measured in surimi subjected to repeated freeze-thaw cycles. In this study, the lipid degradation and lipid oxidation of surimi and the correlation of these processes with different amounts of fat during repeated freeze-thaw process were determined to provide a scientific basis to improve the quality of surimi products for practical production.
Materials and Methods
Fresh grass carp were purchased from a local supermarket of the Carrefour Group, and the live fish were immersed in bags of tap water for transport to the laboratory. Pork back fat was purchased from a local processor, 48 h post-mortem. NH2 -aminopropyl minicolumns were purchased from Agilent Technologies (Santa Clara, CA, USA), N-t-butyl-α-phenylnitrone (PBN) was purchased from Tokyo Chemical Industry, 4-methylumbelliferyl- oleate was purchased from Sigma-Aldrich (St. Louis, MO, USA), and all other chemicals used in this study were analytical grade and purchased from Solabio (Beijing, China).
Surimi was prepared as described previously (Shang et al., 2020a), in accordance with the Regulations on the Administration of Experimental Animals issued by the China State Council in 2017 and the guidance on Treating Experimental Animals developed by China’s Ministry of Science and Technology in 2006. Pork back fat was added to grass carp surimi to achieve final fat content of 0 g/kg, 50 g/kg, 100 g/kg, or 150 g/kg, with the samples designated F0, F50, F100, and F150, respectively. The prepared samples were packed in moisture-impermeable polyethylene bags, sealed, and stored at –18°C. Each week, a set of frozen samples was thawed using flowing water (10°C) and then was frozen and stored at –18°C, the samples underwent six freeze-thaw cycles.
A sample of chopped surimi (2.0 g) was placed in a centrifuge tube, mixed with 25 mL chloroform: methanol (2:1 V/V) solution, and homogenized in an Ultra homogeniser (T25, IKA, Königswinter, Germany) for 1 min. The homogenization liquid was then transferred to a new tube with a plug and the volume was adjusted to 40 mL by addition of chloroform: methanol (2:1 V/V) solution. After standing for 0.5 h, the protein and connective tissue were removed with filter paper, and then normal saline was added (the amount added was estimated based on the optimal extraction conditions of lipids. Finally, a mixture of chloroform: methanol: water=8:4:3), followed by centrifugation at 3,000×g for 15 min. The upper aqueous layer, methanol, ionic impurities, and other solutions were removed, and the remaining liquid was evaporated using a vacuum rotary evaporator (Hei-VAP Advantage MLG3, Heldolph, Schwabach, Germany) in a 40°C water bath to obtain total lipids (samples were then stored at –20°C). Samples (30–60 mg) of the extracted total lipids were dissolved in 5 mL of chloroform: methanol solution (2:1 V/V). Total lipids were fractionated into neutral lipids, free fatty acids, and phospholipids on NH2-aminopropyl minicolumns (100 mg/1 mL, Agilent Technologies) as described by García Regueiro et al. (1994).
Samples (5.0 g) of chopped surimi were homogenized in 25 mL of 50 mM phosphate buffer, pH 7.0, containing 5 mM of Ethylenebis(oxyethylenenitrilo)tetra acetic acid (EGTA). The mixture was homogenized at 25,000 rpm (4×10 s, with cooling on ice) using an Ultra homogenizer (T25, IKA) and then stirred for 30 min in an ice bath. The homogenates were centrifuged at 4°C, 10,000×g for 20 min (CTH 2050R, Thermo Fisher Scientific, Waltham, MA, USA), and then filtered through glass microfiber filters (GF/D, Whatman, Maidstone, UK). The total phospholipase, acid lipase, and neutral lipase activities were determined using a fluorescence spectrophotometer (RF-5301, Shimadzu, Kyoto, Japan) according to Shang et al. (2019).
The peroxide values (PVs) of all samples were determined according to the AOAC method (965.33; AOAC, 1999) and the results were expressed as meq/kg of surimi.
TBARS values were determined as described by Shang et al. (2020a) by measuring the absorbance of each sample at 532 nm. TBARS values were expressed as mg MDA (malondialdehyde equivalents)/kg surimi surimi.
Lipid extraction was performed as described by Shang et al. (2020b) with slight modification. Briefly, 40 g samples were added into 150 mL of petroleum ether and placed on a shaker incubator overnight at 20°C. The mixture was filtered and then the filtrate was evaporated in a vacuum rotary evaporator (Hei-VAP Advantage MLG3, Heldolph) to obtain the lipid sample. Electron spin resonance (ESR) experiments were conducted with a Bruker EMXnano system. Samples were incubated at –20°C and then transferred to room temperature for several minutes to thaw. Next, 50 μL samples were mixed with 5 μL of 70 mg/mL PBN ethanol solution. After mixing, samples were heated at 100°C. ESR detection was done after heating samples for 80 minutes, with the following parameters: microwave frequency 9.646 GHz, power 10 mW, center field 3436 G, sweep width 100 G, modulation frequency 100 kHz, modulation amplitude 2G, 1,000-point resolution, and 30 s sweep time. Radicals were quantified were calculated using Bruker Xenon software with the spin fit and spin count modules.
Lipoxygenase activity was determined according to the methods of Gata et al. (1996) and Fu et al. (2009) with slight modification. Surimi samples (5.0 g) were accurately weighed and then mixed with 3 volumes of 50 mM, pH 7.0 phosphate buffer, containing 1 mM DTT and 1 mM EDTA. After homogenization, the homogenate was stirred in an ice water bath for 30 min, and then centrifuged at 4°C, 15,000×g for 60 min. After centrifugation, the supernatant was filtered with four layers of gauze. In a separate tube, 200 μL sodium linoleate was mixed with 2.9 mL of 50 mM citric acid buffer solution (pH 5.5) and then equilibrated in a 20°C water bath. After the absorbance value at 234 nm had become stable, 0.1 mL enzyme extract was added and the sample was rapidly mixed to uniformity. The absorbance increase in 1 min was measured at 20°C and 234 nm. One enzyme activity unit was defined as an increase of 1 in absorbance per gram of enzyme protein per minute.
The means and SDs of triplicate measurements were determined. To assess statistical significance, SPSS 16.0 was used for one-way analysis of variance (ANOVA) and two-way ANOVA for single factor analysis and the interaction of freeze-thaw cycle and fat content, respectively. Statistical differences between different treatments were assessed by Tukey’s multiple range test, using a significance limit of p<0.05. Additionally, to evaluate the relationships between variables, Pearson’s two-tailed correlation analysis was performed by SPSS 16.0.
Results and Discussion
The lipid composition was determined for grass carp surimi as shown in Table 1. Samples subjected to freezing and thawing cycles exhibited changes in the neutral lipid content of 74.26%–77.40%. Compared with neutral lipids, more significant changes were observed for the levels of phospholipids and free fatty acids. Freeze-thaw treatment resulted in decreased phospholipid content and increased free fatty acid content due to the lipolysis of lipids by lipase (Qiu et al., 2013). In the same freeze-thaw cycle, the content of neutral lipids changed little with the increase of pork back fat, while the content of phospholipids decreased and the content of free fatty acids increased. This shows that differences in the amount of added fat affects lipid composition in surimi.
F represents surimi without freeze-thaw cycle, C1–C6 represent surimi with 1–6 freeze-thaw cycles respectively. F0, F50, F100, and F150 represent surimi with 0 g/kg, 50 g/kg, 100 g/kg, and 150 g/kg of pork back fat respectively.
For samples subjected to different numbers of freezing and thawing cycles, there was no correlation between neutral lipid content, free fatty acid content, and phospholipid content in surimi containing 0%, 5%, 10%, and 15% pork back fat, but there was a significant negative correlation between free fatty acid content and phospholipid content. This indicated that the observed increase of free fatty acids was mainly due to the decomposition of phospholipids, and may also be related to neutral lipids, a finding that is consistent with the results of a previous study of the effects of pork back fat addition by Huang et al. (2014). For samples subjected to the same number of freeze-thaw cycles, there was no correlation of the contents of neutral lipids, free fatty acids, and phospholipids with different contents of pork back fat, except for a negative correlation between free fatty acids and phospholipids at the 6th freeze-thaw cycle.
Lipases (including total phospholipases, acid lipases, and neutral lipases) in fish tissues are conducive to lipid hydrolysis (Toldrá, 2006), and lipase activities in the processing of meat products and aquatic products can vary with different raw materials, varieties of the same raw material, lipase types, or processing conditions (Huang et al., 2014). However, few studies have examined the effect of freeze-thaw treatment on lipases. In our study, neutral lipase activity was the highest, followed by total phospholipase activity, and acid lipase showed the lowest activity (Table 2). Repeated freezing and thawing can destroy muscle cells due to the presence of ice crystals, resulting in the release of lipases (Sun et al., 2019). However, the lipase activity did not increase but decreased maybe due to the inhibition of lipase activities (Leygonie et al., 2012). After six freeze-thaw cycles, the activity of total phospholipase, acid lipase, and neutral lipase was still retained, with activities of 34.36%–41.21%, 7.64%–13.02%, and 4.75%–13.72%, respectively, indicating that lipase continued to degrade lipid in the whole process. For the same freeze-thaw cycle, the total phospholipase, acid lipase, and neutral lipase activities increased with the increase of pork back fat content, which was consistent with the results of lipid composition.
F represents surimi without freeze-thaw cycle, C1–C6 represent surimi with 1–6 freeze-thaw cycles respectively.
F0, F50, F100, and F150 represent surimi with 0 g/kg, 50 g/kg, 100 g/kg, and 150 g/kg of pork back fat respectively.
A–D Means within pork back fat content with different superscript letters are significantly different (p<0.05).
Hydroperoxide is the primary oxidation product of lipid oxidation and can be evaluated as the PV. The PVs of grass carp surimi samples with different amounts of fat content were determined and the results are shown in Table 2. Subjected to repeated freezing and thawing, the PVs of surimi generally showed an increasing trend, a result that was consistent with the finding of Nikoo et al. (2015). With increased pork back fat content, the PV of surimi first increased and then decreased. This result differs from the finding of Pan et al. (2021) who repeatedly freeze-thawed quick-frozen pork patty samples containing different amounts of pork back fat and found that PV first increased and then decreased with the increase of freeze-thaw cycles, and PV increased with the increase of pork back fat content.
TBARS is often measured to assess the degree of lipid oxidation. As shown in Table 2, TBARS increased significantly (p<0.05) with the increase of freeze-thaw cycle. Ice crystals that form during repeated freezing and thawing can lead to muscle tissue damage, which may lead to the release of pro-oxidants (Leygonie et al., 2012) as well as lipases, proteases, nucleases, and oxidases in cells (Sun et al., 2019), thus accelerating lipid oxidation.
For samples subjected to the same numbers of freeze-thaw cycles, TBARS increased significantly with the increase of fat content (p<0.05). There was also a significant positive correlation between fat content and lipid oxidation, a finding that is consistent with the results of Liu et al. (2018) and Utrera et al. (2014).
Lipid oxidation first produces free radicals, earlier than peroxide (Jiang et al., 2020). Generally, free radicals can be detected and quantified by ESR spectroscopy, and correlation between free radical formation and stability of lipid oxidation has been confirmed for several foods (Jensen et al., 2005). In our study, the ESR intensity and free radical content of the samples were measured and the results are shown in Fig. 1 and Table 2. We also studied the correlation between free radical content and other lipid oxidation indexes. With repeated freezing and thawing, free radicals, PV, and TBARS were positively correlated (free radicals and PV were not correlated in the surimi samples with 5% pork back fat). This result was not consistent with the study of Jiang et al. (2020), who reported no correlation between free radical formation and PV in edible oil oxidation. The free radical content and lipoxygenase activities were positively correlated (except for in surimi samples with 0% pork back fat). For samples treated with the same numbers of freezing and thawing cycles, there was no correlation between free radical content and PV, TBARS, or lipoxygenase.
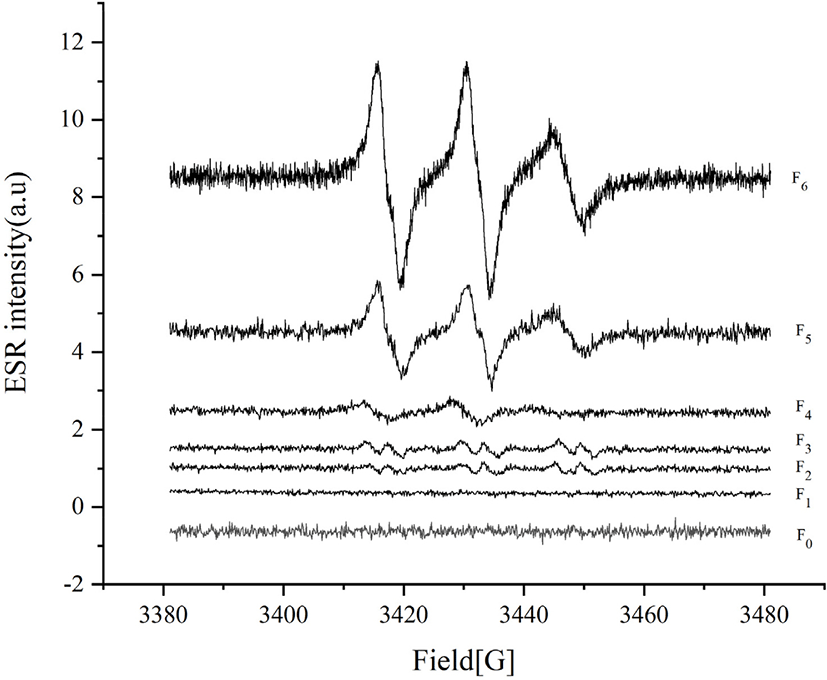
Lipoxygenase (LOX) can induce the oxidation of polyunsaturated fatty acids to produce unstable hydroperoxides (Hsieh et al., 1988). Stodolnik et al. (2005) found that LOX activity of mackerel increased during freezing, leading to increased lipid oxidation. In our study, LOX activity increased with the increase of freeze-thaw times. The activity of LOX first increased and then decreased with the increase of pork back fat. This result is consistent with the observed PV trend.
Table 3 shows the interaction of freeze-thaw cycle and fat content. From Table 3, we can find that the interaction of freeze-thaw cycle and fat content is significant (p<0.05) on all index values except the neutral lipid content index (p>0.05).
During processing and storage, the hydrolysis of lipids by lipase produces free fatty acids. Lipoxygenase generates peroxides from these free fatty acids, and the resulting peroxides are further decomposed into aldehydes, ketones, and acids (Jin et al., 2011; Toldrá, 2006). Lipid composition, lipolysis, and lipid oxidation were measured in the treated samples and the results are shown in Table 4 and described in detail below.
For samples subjected to repeated freezing and thawing, there was no correlation between neutral lipid content and the three lipase activities in surimi samples with 0%, 5%, 10%, and 15% added fat (p>0.05). There was a significant negative correlation (p<0.05) or extremely significant negative correlation (p<0.01) between free fatty acids and the three lipase activities in all samples, and a significant negative correlation (p<0.05) or extremely significant negative correlation (p<0.01) between phospholipids and the three lipase activities, indicating that lipase changed phospholipids into free fatty acids (Qiu et al., 2013).
For the same freeze-thaw cycle, samples prepared with different contents of pork back fat were compared. The results showed that, for the 0–3 freeze-thaw cycles, neutral lipids were negatively correlated with lipase activities and free fatty acids were positively correlated with lipase activities, but phospholipids were not correlated with lipase activities. In the 4-6 freeze-thaw cycles, phospholipids were negatively correlated with lipase activities, free fatty acids were positively correlated with lipase activities, and neutral lipids were not correlated with lipase activities. These data and the results in Table 1 indicate that neutral lipids are the main source of free fatty acids in the early stage of freeze-thaw, while phospholipids are the main source of free fatty acids in the late stage.
For samples submitted to repeated freezing and thawing, there was no correlation (p>0.05) between the neutral lipid content and the four related lipid oxidation indicators (free radical content, PV, TBARS, and lipoxygenase) with surimi with 0%, 5%, 10%, and 15% added pork back fat. However, there was a positive correlation between the free fatty acid content and these four lipid oxidation indicators (p<0.05) except for the PV for surimi with 0% added fat. There was a negative correlation between phospholipid content and lipid peroxidation (p<0.05) for all samples, except for the PV of surimi supplemented with 0% fat, suggesting that lipid degradation can promote lipid oxidation (Yang et al., 2005). For samples subjected to the same number of freeze-thaw cycles, there is little effect of different additions of pork back fat and little correlation between lipid composition and the four measured indicators of lipid oxidation (free radical content, PV, TBARS, and lipoxygenase).
Conclusion
The degree of lipolysis, lipid oxidation and its correlation in surimi samples containing different amounts of fat and subjected to repeated freezing and thawing were studied for the first time. During repeated freezing and thawing, lipolysis can promote lipid oxidation, and surimi products with high fat content are more vulnerable to oxidative damage. Therefore, to ensure high quality surimi, appropriate amounts of fat should be added during processing and temperature fluctuations due to freezing and thawing should be avoided during storage.