Introduction
Freezing is the most widely used storage method for aquatic products, maintaining the quality of aquatic products and extending product shelf life. However, if the cold chain system is not standardized, aquatic products may experience freeze-thaw cycles during steps of storage, transportation, retail and sales (Srinivasan & Hultin, 1997). When the number of freeze-thaw cycles increases, the edible quality of aquatic products will become lower. In the 2015 study of Nikoo et al., Japanese sea bass surimi went through six freeze-thaw cycles to compare peroxide value (PV), thiobarbituric acid reactive substances (TBARS), protein carbonyl and total sulfhydryl content before and after the cycle, it is found that PV, TBARS and protein carbonyl content increased and total sulfhydryl content decreased with the increase of the number of freeze-thaw cycles (Nikoo et al., 2015). In 2019, Nikoo et al. also found that with increasing cycle numbers, there was an increase in the protein carbonyl content of Asian black bass surimi, and a decrease in total sulfhydryl content and protein solubility (Nikoo et al., 2019). The observed changes of these indexes indicated a decrease in the quality of surimi with increasing cycle numbers. Sriket et al. (2017) pointed out that repeated freezing and thawing of Sawai (Pangasianodon hypophthalmus) makes an increase in the degree of lipid oxidation, and a decrease in lightness. Overall, the quality problems of aquatic products resulting from repeated freezing and thawing are increasingly concerned by processors and consumers.
Consumption of surimi products is becoming more and more popular. During the processing of some surimi products (such as fish slide and kamaboko), pork back fat may be added into products to improve the flavour, smoothness, and juiciness (Chojnicka et al., 2009). However, the increased fat content may not only contribute to improve the taste, but may also promote lipid and protein oxidation, thus affecting the food quality and nutritional value of the product (Pietrowski et al., 2011; Shi et al., 2014). In this study, fresh grass carp (Ctenopharyngodon idella) was used as raw material to prepare surimi with different fat content by adding salt and different proportion of pork back fat. The water distribution, TBARS, color, myoglobin oxidation, heme iron and non-heme iron content of samples were determined to analyze the effects of different fat content on lipid oxidation and myoglobin oxidation during repeated freeze-thaw cycles of grass carp surimi.
Materials and Methods
The selected fresh grass carp are bought in a supermarket-Carrefour supermarket. All the fish are soaked in tap water in bags to keep them fresh, and then transported to the laboratory. Pork back fat was purchased at the local market, 48 h post-mortem. Heme was purchased from Sigma-Aldrich (St. Louis, MO, USA), and all the chemicals used in this study were purchased from Solabio at analytical grade.
The State Council issued the regulations on the Administration of Experimental Animals in 2017, and China’s Ministry of Science and Technology compiled the guidance on the treatment of Experimental Animals in 2006, based on these two documents, using sticks to hit all fish on the head, causing their death. Then the scales and internal organs were removed, the head was cut off and the fish was cut into thin slices, and washed for 5 min with cold water (5°C) and then were chopped for 120 s in a food processor (HR3868, Philips, China). 25 g/kg of salt was added to surimi and stirred for a duration of 2 min. Then pork back fat was added and stirred for a duration of 3 min. The final fat content of grass carp surimi was 0 g/kg, 50 g/kg, 100 g/kg, and 150 g/kg respectively, and the samples were named F0, F50, F100, and F150 respectively. These samples were packed in moisture impermeable polyethylene bags, sealed and stored at –18°C for each cycle. For each week, a set of frozen samples was thawed at flowing water. The frozen samples were subjected to 6 freeze-thaw cycles. A weekly analysis of the samples was performed over six weeks with thawing of samples.
LF-NMR relaxation measurements were done according to the method of Aursand et al. (2008), which is slightly modified. Sample (1×1×1 cm) was added to the cylindrical glass tube and Carr-Purcell-Meiom-Gill sequence was used to measure relaxation time of T2. The threshold value used for T2 measurement is 150 μs. The data of 4,000 echoes were collected repeatedly through 12 scans. The next repetition time between the scan and the scan is 4,000 ms. Through the use of Nuimag’s Multi Exp Inv Analysis software to make a multi-exponential fitting analysis, a total of 3 relaxation times (T2b, T21, and T22) were obtained, and the corresponding percentages of different types of groups (P2b, P21, and P22) were also obtained.
Krzywicki (1982) and Shang et al. (2020) method was used to extract myoglobin from grass carp surimi. Five g of samples were weighed, followed by addition of 50 mL phosphate buffer (40 mmol/L, pH 6.8, 4°C). With the application of Ultra homogenizer (T18, IKA, Königswinter, Germany), samples were homogenized under the condition of 10,800 rpm for 20 s and placed in an ice bath for 60 min, then the frozen centrifuge (GL20M, Herixi, Changsha, China) was used in 4°C conditions, 10,000×g was centrifuged for half an hour. Whatman No. 1 filter paper was used to filter the supernatant, and UV visible spectrophotometer (UV2559, Shimadzu, Kyoto, Japan) was used to scan at full wavelength and record the absorbance at 525 nm, 545 nm, 565 nm, and 572 nm. The proportions of the three forms of myoglobin were calculated as follows:
where R1 = A572/A525, R2 = A565/A525 and R3 = A545/A525.Beltran et al. (2003) method was used to measure TBARS. Combined with the absorbance of different samples at 532 nm, the TBARS values were calculated. At the same time, the new samples were acidified by 1,1,3,3-tetraethoxypropane, and the standard curve of malondialdehyde (MDA) (8–50 nmol) was constructed. The TBARS is expressed as mg MDA×kg−1 surimi.
According to the color of grass carp surimi, redness (a*), yellowness (b*), and lightness (L*) were evaluated by the use of Spectro-colorimeter (Miniscan EZ, HunterLab, Reston, VA, USA). Six measurements of these coordinates were performed for each of the three replicates.
The methods of Shang et al. (2020) were used to measure the content of heme iron with slightly modified. First, a 10 g grass carp surimi sample was added into 20 mL acidified acetone (80% acetone, 18% water and 2% HCl). Then, with the use of Ultra homogenizer (T18, IKA), the mixture was homogenised under the condition of 15,000 rpm for 15 s and remained in the dark state for 1 hour under the condition of 4°C. Then it was centrifuged at 10,000×g (4°C) for 10 min through a frozen centrifuge (GL20M, Herixi). After precipitation, the soluble substance is obtained and passed through a fiberglass filter (GF/A, Whatman, Maidstone, UK). The filtrate’s absorbance was measured at 640 nm. The standard curve established by using hematin and the heme iron content was 0.0882 times of hematin content.
The method proposed by Ma et al. (2016) was applied to the measurement of non-heme iron with slightly modified. Firstly, a 5 g grass carp surimi sample was added into 15 mL of 0.1 mol/L citric acid-phosphate buffer (pH 5.5). The mixture was homogenized for 15 s by Ultra homogenizer (T18, IKA) at 15,000 rpm and filtered the supernatant. Then the 1 mL 2% ascorbic acid and 3 mL homogenate were mixed together and incubated at 20°C for a duration of 20 min. 2 mL trichloroacetic acid (11.3%) was then added, mixed together, and centrifuged at a high speed under the condition of 10,000×g (10°C) using a frozen centrifuge (GL20M, Herixi) for 10 min. The transparent supernatant of 3 mL was then transferred into the test tube, which was effectively mixed with the ferrozine chromogenic agent of 0.3 mL and 10% ammonium acetate of 1.2 mL. The mixture was filtered by the use of a fiberglass filter (GF/A, Whatman), and then the absorbance was measured at 562 nm. All iron determination processes are carried out in a dark state.
Results and Discussion
There are three types of water in the muscle. The shortest relaxation time 1–10 ms (T2b) represents water firmly bound to macromolecules. The intermediate relaxation time 20–100 ms (T21) represents immobilized water. Of all the types, the third type had the most lasting relaxation time, 200–1,000 ms (T22), which was corresponded to water outside the myofibril (Zang et al., 2017). Immobilized water content is very important in grass carp surimi, and water-holding capacity is greatly affected by this part of water (Qin et al., 2017). Therefore, it is necessary to study the relaxation time and proportion of immobilized water. In our study, T21 increased in the first two cycles which might be related to the salt-induced swelling of myofibers (Aursand et al., 2009) and the expansion of space between myofilaments due to increased protein electrostatic repulsion (Erikson et al., 2004). From cycle 3 to cycle 6, T21 and p21 decreased significantly with the increase of freeze-thaw cycles (Table 1), due to protein denaturation, muscle fiber contraction, and water flow to the extracellular space (Ali et al., 2015). With increasing fat content, T21 decreased significantly and p21 increased significantly, suggesting that fat is conducive to the formation of the fine three-dimensional-structured network of surimi, making more water bound in myofibrillar protein (Yang et al., 2015).
A–D Means within pork back fat content with different superscript letters are significantly different (p<0.05).
Myoglobin belongs to a kind of globular heme protein, which exists in the animal-derived foods and products. It affects the muscle color to a great extent, and is greatly affected by its redox state and concentration (Chaijan, 2008). In the presence of oxygen, deoxymyoglobin and oxymyoglobin were oxidized to metmyoglobin. Compared with mammalian myoglobin, fish myoglobin is easier to oxidize (Haard, 1992). The metmyoglobin contents in grass carp surimi were measured and shown in Table 1. In the same freeze-thaw cycle, with the gradual increase of fat content, the content of metmyoglobin in grass carp surimi increased significantly. Moreover, with more and more freeze-thaw cycles, the content of metmyoglobin increased significantly in grass carp surimi without pork back fat. Freeze-thaw oxidizes deoxymyoglobin and oxymyoglobin to form metmyoglobin, which is consistent with the conclusion of Jiang et al. (2019). However, in the samples with different amounts of pork back fat, the content of metmyoglobin increased at first and then decreased. which may reflect the reduction of metmyoglobin to oxymyoglobin by ferrimyoglobin reductase (Wongwichian et al., 2015).
The absorption spectra of myoglobin in all surimi samples were measured from 380 to 450 nm, as shown in Fig. 1 and Table 1. With the fat content increased, the absorption peak of porphyrin showed blue shift. With the increase of freeze-thaw times, porphyrin absorption peak also blue shifted, which shifts from 409 nm to 402 nm. This result is consistent with Wongwichian et al. (2015) and Wang et al. (2018), which suggested that oxidation reaction was caused by repeated freeze-thaw cycles and increased fat content. The polypeptide chain of myoglobin consists of 153 amino acids, globin and an artificial heme group, and an iron (II) protoporphyrin-IX complex (Hayashi et al., 1998). Porphyrin compounds have a strong absorption peak at 420 nm because the haem moiety and apomyoglobin interact with each other. Therefore, the porphyrin absorption peak can be used to monitor the unfolding of heme protein. When the peak disappears, it indicates that the heme protein is damaged or the porphyrin part is separated from globin (Benjakul & Bauer, 2001; Wongwichian et al., 2015). In this study, when the number of freeze-thaw cycles gradually increases, the height of porphyrin absorption peak begins to decrease, which is consistent with changes of heme iron and non-heme iron.
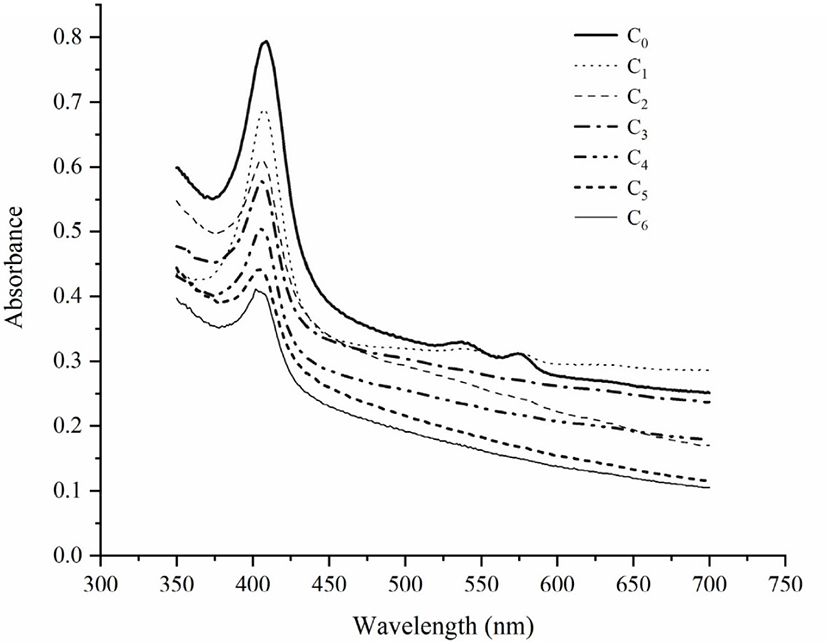
TBARS is a common method used to determine MDA, the final oxidation product of which is unsaturated fatty acid (Karoui & Blecker, 2011). The states of MDA are relatively stable, and can reflect the degree of lipid oxidation to a certain extent (Guillén-Sans & Guzmán-Chozas, 1998). Benjakul & Bauer (2001) pointed out that freeze-thaw of muscle tissue led to the accumulation of TBARS in a short time, which was attributed to the destruction of cell membrane caused by ice crystals and the subsequent release of oxidants, especially heme iron. With the increase of freeze-thaw times, the TBARS of grass carp surimi increased significantly (Table 2), which was consistent with the results of Wang et al. (2015). When the fat content increased, TBARS increased significantly, with a maximum value of 2.87 mg/kg, which is in the allowable range of surimi: MDA content greater than 2–3 mg/kg confers bad flavour to the meat (Ferreira et al., 2016).
A–D Means within pork back fat content with different superscript letters are significantly different (p <0.05).
The freeze-thaw cycle results in tissue damage and protein denaturation, resulting in a decrease in total iron and heme iron in fish (Sriket et al., 2007; Turhan et al., 2006). With the increase of freeze-thaw times, heme iron in grass carp surimi decreased significantly, while non-heme iron increased significantly (Table 2). This may be due to the destruction of iron, resulting in the release of free iron (Wongwichian et al., 2015). When the fat content increased gradually, the heme iron content in grass carp surimi decreased significantly, and the non-heme iron content increased significantly, which was closely related to fat oxidation (Maqsood & Benjakul, 2010).
After freezing and thawing many times, the lightness of grass carp surimi gradually disappeared and L* decreased (Table 2). There are two explanations for these changes. First, the carbonyl compounds formed by oxidation may react with amino compounds such as amines and amino acids to generate dark substances, resulting in decreased lightness (Giri et al., 2010). Second, the catalytic oxidation of iron and copper in fish can promote deepening of the color (Tokur & Korkmaz, 2007). The a* value of grass carp surimi is low and negative, and the b* value is relatively high, which indicates that the color of grass carp surimi is yellow and green. With the increase of freeze-thaw times, the value of a* increases, which may be related to the decrease of heme protein (Leygonie et al., 2012) and the increase of metmyoglobin (Canto et al., 2012). Shi et al. (2014) found that adding vegetable oil will increase the L* and a* values of surimi, and the b* value was affected by the color of vegetable oil itself. In our study, in the same freeze-thaw cycle, with increasing fat content, there were increases in L* values and a* values, and decreases in b* values.
Table 3 was the interaction of freeze-thaw cycle and fat content. From Table 3, we can find that the interaction of freeze-thaw cycle and fat content have significant effect on T21, metmyoglobin content, TBARS, heme iron content, a* and b* (p<0.05), and the interaction of freeze-thaw cycle and fat content have no significant effect on p21, non-heme iron content and L* (p>0.05).
Conclusion
The effect of different fat content on lipid and myoglobin oxidation of grass carp surimi under multiple freeze-thaw cycles was investigated. The freeze-thaw cycle contributes to lipid oxidation, myoglobin oxidation and the conversion of heme iron to non-heme iron, which eventually leads to a decrease of immobilised water content in muscle and a decrease of color stability. Therefore, to prevent these negative effects on the quality of grass carp surimi, temperature fluctuation must be avoided during freezing, transportation, and storage. When fat was added, the water distribution, TBARS, color, metmyoglobin, heme iron and non-heme iron content of grass carp surimi was adversely impacted. Increased fat content promoted the binding of water in myofibrillar protein and improved the lightness of grass carp surimi, but resulted in the aggravation of the degree of lipid and myoglobin oxidation. Overall, the results suggest that careful selection of the appropriate fat content is required for the optimal processing of surimi products.