Introduction
Staphylococci are frequent inhabitants of the normal microbiota of skin and mucous surfaces in humans and animals (Davis, 1996). Although less frequent than the coagulase-positive staphylococci such as S. aureus, coagulase-negative staphylococci (CoNS) can cause many nosocomial and community-associated infections including skin and soft tissue infections, urinary tract infections, endocarditis, and blood stream infections (Piette and Verschraegen, 2009). Moreover, several recent studies have reported the potential role of non-aureus staphylococci (NAS) in transmission of antimicrobial resistance by acting as a reservoir for antimicrobial resistance genes (Archer and Niemeyer, 1994; Nemeghaire et al., 2014a).
Fusidic acid (FA) is a bacteriostatic steroid antibiotic originated from Fusidium coccineum, previously used to treat staphylococcal skin infections since the early 1960s (Godtfredsen et al., 1962). It targets elongation factor G (EF-G) that functions as ribosomal translocase and interact with ribosomal recycling factor to release ribosomal complexes (Fernandes, 2016). However, FA resistance can occur due to spontaneous mutations in fusA, which encodes EF-G (Turnidge and Collignon, 1999). Point mutations in fusE, encoding ribosomal protein L6, are also associated with FA resistance in staphylococci (Norström et al., 2007). In addition, FusB-family proteins bind to EF-G and protect them from FA binding (O’Neill and Chopra, 2006). The FusB-family proteins are produced by the fusB, fusC, fusD, and fusF genes and frequently mediate low-level resistance to FA (Fernandes, 2016). These fusB-family genes have been reported in S. aureus and NAS isolates, either carried on a plasmid, phage-associated resistance islands, or staphylococcal cassette chromosome (SCC) (Chen et al., 2015; O’Neill and Chopra, 2006; O’Neill et al., 2007). It has been well known that S. saprophyticus and S. cohnii subsp. urealyticus possess fusD and fusF genes for their intrinsic FA resistance (Chen et al., 2015; O’Neill et al., 2007).
The significance of food-producing animals as carriers of foodborne zoonotic pathogens and antimicrobial resistance genes has been demonstrated in many countries including Korea (Hung et al., 2015; Nam et al., 2011; Nemeghaire et al., 2014a). In contrast to coagulase-positive S. aureus, well recognized as a major causative pathogen of staphylococcal food poisoning and antimicrobial resistance, occurrence of NAS in foods of animal origin, their antimicrobial resistance phenotypes, and the genetic factors associated with the resistant phenotypes have not been well investigated. Therefore, we aimed to investigate i) the profiles of NAS in pork and carcass samples collected from retail markets and slaughterhouses in Korea, ii) the antimicrobial resistance phenotypes of the staphylococcal isolates, and iii) the occurrence and distribution of fusB-family genes (fusB, fusC, fusD, and fusF), and iv) the point mutations in fusA genes by sequencing analyses.
Materials and Methods
We obtained a total of 578 swab or pork samples from seven slaughterhouses (311 carcass samples) and 35 retail markets (267 pork samples) across eight Korean provinces in 2018. Slaughterhouse carcass samples were obtained from a single visit at seven different slaughterhouses: Gyeonggi (46 swabs), Gangwon (46 swabs), Chuncheong (46 swabs), Jeolla (two slaughterhouses, 40 and 42 swabs), and Gyeongsang (two slaughterhouses, 45 and 46 swabs). Each carcass swab was prepared on an area of (10×10 cm) per site on the back and chest of pig carcasses within 8 h of slaughter. Fresh pork samples were collected from four to five retail markets in each province. All samples were kept at 4°C and processed for isolation of staphylococci within 24 h of collection.
Swab samples from slaughterhouses were inoculated into 6 mL of tryptic soy broth (TSB; Difco Laboratories, Detroit, MI, USA) containing 10% sodium chloride (NaCl) for enrichment at 37°C. Each pork sample (25 g) was homogenized in 225 mL of 10% NaCl-TSB. After overnight incubation, 15 μL aliquots of the pre-enriched NaCl-TSB cultures were streaked onto Baired-Parker agar (BPA; Difco Laboratories) supplemented with potassium tellurite and egg yolk, and then grown at 37°C for up to 48 h. Next, up to two presumptive staphylococcal colonies from each plate were re-streaked on BPA plate for identification. For genomic DNA isolation, individual isolates were inoculated into fresh TSB, cultured at 37°C for 18–24 h, and bacterial cell pellets were subjected to the Genmed DNA extraction kit (Genmed, Seoul, Korea) according to the manufacturer’s protocols. Identification of staphylococcal species was performed using matrix-assisted laser desorption/ionization time-of-flight mass spectrometry (MALDI-TOF/MS; Bruker Daltonics GmbH, Bremen, Germany) and 16S rRNA sequencing. For bacterial identification using MALDI-Biotyper Realtime Classification system, presumptive staphylococci were placed on a target plate coated with specific energy-absorbent agent, the matrix. The sample within the matrix was then ionized in an automated mode with a laser beam as recommended by the manufacturer. Next, peptides in bacterial sample converted into protonated ions and the peptide mass fingerprints were used to identify bacterial species based on the spectral database (MALDI Biotyper 3.1, Bruker Daltonics GmbH). Score values ≥2.0 were used for an identification of staphylococcal species. For 16S rRNA sequencing analyses, 16S rRNA genes were PCR-amplified using a universal primer set (16S_27F: 5’-AGA GTT TGA TCC TGG CTC AG-3’ and 16S_1492R: 5’-TAC GGY TAC CTT GTT ACG ACT T-3’) (Mendoza et al., 1998). PCR amplifications were performed as follows: Denaturation at 94°C for 30 s followed by 28 cycles of 94°C for 30 s, 52°C for 30 s, and 72°C for 1 min. After a final 10 min extension at 72°C, the samples were purified using PCR purification kit (Bionics, Seoul, Korea), and then sequenced at Bionics.
Standard disc diffusion methods were used to determine the antimicrobial susceptibility of each isolate according to Clinical and Laboratory Standards Institute’s (CLSI) recommendations for the following antimicrobial agents: penicillin (PEN, 10 μg), ampicillin (AMP, 10 μg), cefoxitin (FOX, 30 μg), chloramphenicol (CHL, 30 μg), clindamycin (CLI, 2 μg), erythromycin (ERY, 15 μg), FA (10 μg), ciprofloxacin (CIP, 5 μg), mupirocin (MUP, 200 μg), trimethoprim/sulfamethoxazole (SXT, 1.25/23.75 μg), tetracycline (TET, 30 μg), rifampicin (RIF, 5 μg), gentamycin (GEN, 10 μg), quinupristin/dalfopristin (SYN, 15 μg). The minimum inhibitory concentrations (MICs) to FA (Liofilchem, Roseto degli Abruzzi, Italy) and oxacillin (OXA; bioMérieux, Marcy l’Etoile, France) were determined by standard E-test. OXA E-test was performed on MH BBL II agar (Becton Dickinson, Sparks, MD, USA) supplemented with 2% NaCl. The breakpoint for FA resistance (>1 μg/mL) was based on the European Committee on Antimicrobial Susceptibility Testing (EUCAST) (EUCAST, 2021) guidelines. The S. aureus ATCC 25923 strain was included as a reference for the antimicrobial susceptibility analyses.
All the strains showing resistance to OXA or FOX were examined for the presence of mecA gene using the PCR method as described previously (Geha et al., 1994). The mecA positive staphylococcal strains were subjected to SCCmec typing as previously described (Kondo et al., 2007). A series of multiplex PCR reactions were employed to amplify mec regulatory elements (mec) and chromosomal cassette recombinase (ccr) genes. The combinations mec complexes and ccr types were used to determine the SCCmec types of the staphylococcal strains. The PCR was preceded as previously described (Kondo et al., 2007).
The carriage of acquired FA resistance genes fusB,fusC, fusD, and fusF were detected by PCR methods as described before (Chen et al., 2010; Chen et al., 2015; McLaws et al., 2008). The primers used to amplify fusB-family genes are listed in Table 1. For detection of fusB-family gene homologues in M. sciuri (previously S. sciuri) strains, a specific primer set was designed based on the published sequences of 7 M. sciuri strains (https://www.ncbi.nlm.nih.gov) carrying the fusB/C-family genes (Table 1). The PCR conditions for detecting fusB/C in M. sciuri were as follows: Denaturation at 95°C for 2 min, followed by 28 cycles of denaturation at 94°C for 35 s, annealing at 53°C for 40 s, extension at 72°C for 45 s, and final extension at 72°C for 10 min.
Primer name | Sequence (5’→3’) | Product size (bp) | Target gene | References |
---|---|---|---|---|
BF | CTA TAA TGA TAT TAA TGA GAT TTT TGG | 431 | fusB | (McLaws et al., 2008) |
BR | TTT TTA CAT ATT GAC CAT CCG AAT TGG | |||
CF | TTA AAG AAA AAG ATA TTG ATA TCT CGG | 332 | fusC | (McLaws et al., 2008) |
CR | TTT ACA GAA TCC TTT TAC TTT ATT TGG | |||
B/C-F | CTT AAA AGC TAC GTC GTC CCA | 299 | fusB/C | This study |
B/C-R | CCA TCA CCT TTA GAT TTC GTC GTA | |||
AF | GCT CAT TAC CGT TGG TAA GAT AGA A | 2.5k | fusA | This study |
AR | TTG GCA TGT GTT TTT GAG CGA | |||
A-S1 | TTA ATT GAA GCT GTT GCT GA | Sequencing only | ||
A-S2 | TGC ACA AGT TCA AGG TAA ATT CTC | Sequencing only | ||
DF | AAT TCG GTC AAC GAT CCC | 465 | fusD | (Chen et al., 2010) |
DR | GCC ATC ATT GCC AGT ACG | |||
FF | CTA AAA TAG ACA TTT ATC AGC AG | 427 | fusF | (Chen et al., 2015) |
FR | GGT ATA TTG TCC ATC ACC AG |
To detect point mutations within fusA genes in FA resistant isolates, DNA sequencing analyses were performed. Using the specific primer set (AF and AR) as shown in Table 1, fusA gene was amplified from genomic DNA samples purified from S. epidermidis and M. sciuri strains. The PCR amplicons were sequenced with AF and two additional primers A-S1 and A-S2 at Bionics (Seoul, Korea). The fusA sequence data were then compared with the published sequences of S. epidermidis (GenBank: NZ_CP035288.1) and M. sciuri (GenBank: CP071138.1).
Except one S. hyicus strain, whose MLST scheme has not yet been developed, MLST was performed on all S. aureus, S. epidermidis, and M. sciuri isolates as described previously (Enright et al., 2000; Schauer et al., 2021; Thomas et al., 2007). For MLST analyses, internal fragments of seven housekeeping genes from each strain were PCR amplified and sequenced. The seven genes amplified from each species of staphylococci are: arcC, aroE, gmk, glpF, pta, tpi, and yqiL for S. aureus (Enright et al., 2000); arcC, aroE, gtr, pyrR, mutS, yqiL, and tpiA for S. epidermidis (Thomas et al., 2007); ack, aroE, glpK, ftsZ, gmk, pta1, and tpiA for M. sciuri (Schauer et al., 2021). The alleles and sequence types (STs) of each staphylococcal species were assigned according to the MLST databases (https://www.pubmlst.org/).
Results
As presented in Table 2, 44 staphylococci (44/311, 14.1%) of four different species were isolated from slaughterhouse carcass samples, and only three strains of M. sciuri (3/267, 1.1%) were isolated from retail pork samples obtained over the 10-month study period. The most frequent staphylococci identified in the carcasses were M. sciuri (previously S. sciuri), comprising ~66% (29/44) of the staphylococcal isolates from slaughterhouse samples. While four different species (S. aureus, S. hyicus, S. epidermidis, and M. sciuri) were identified in the slaughterhouse samples, only three strains of M. sciuri were cultured from retail pork meat samples. All of the 47 isolates used in this investigation were obtained from different carcass or meat samples.
MLST analyses of the S. aureus and S. epidermidis isolates revealed four and six different STs, respectively, with four non-typeable S. epidermidis strains (Fig. 1). The 32 strains of M. sciuri were assigned to three different STs: ST63 (n=10, 31.3%), ST30 (n=8, 3%), and ST96 (n=1, 3.1%) with 13 non-typeable strains under the current M. sciuri MLST scheme.
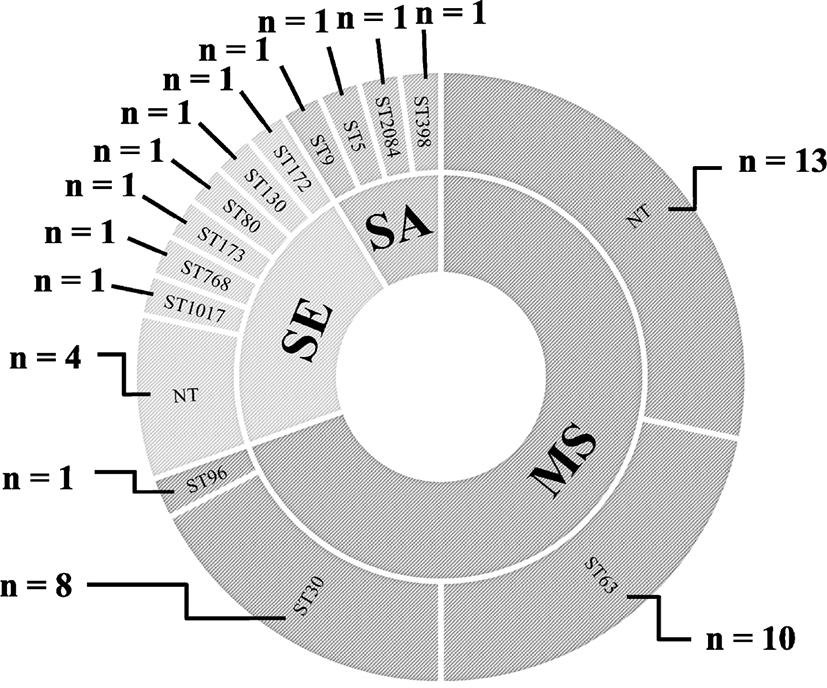
Seven methicillin-resistant staphylococci, two methicillin-resistant S. aureus (MRSA) and five methicillin-resistant S. epidermidis (MRSE) strains, were identified among the 47 staphylococcal isolates, indicating 14.9% methicillin resistance prevalence (Table 2). All seven methicillin-resistant staphylococcal strains were mecA positive and exhibited resistant phenotype to OXA, OXA MIC ≥4 μg/mL (S. aureus), OXA MIC ≥0.5 μg/mL (S. epidermidis) (Table 3). Each of the five MRSE strains were assigned to five different STs of ST172, ST130, ST80, ST173, and ST768. The two MRSA strains were ST398 with SCCmec V and ST2084 with SCCmec IV, respectively (Table 2). Although all 32 M. sciuri strains showed OXA MICs of ≥0.5 μg/mL, none of the strains were FOX-resistant (Table 3). Furthermore, all the M. sciuri strains were negative for the mecA gene.
MICs, minimum inhibitory concentrations; OXA, oxacillin; TET, tetracycline; FA, fusidic acid; SCC, staphylococcal cassette chromosome; MLST, multi-locus sequence type; MRSA, methicillin-resistant S. aureus; AMP, ampicillin; FOX, cefoxitin; PEN, penicillin; CHL, chloramphenicol; CIP, ciprofloxacin; TET, tetracycline; NA, not applicable; MSSA, methicillin-susceptible S. aureus; MUP, mupirocin; ERY, erythromycin; SXT, trimethoprim/sulfamethoxazole; NT, not-typable stain; CLI, clindamycin; S. epidermidis, Staphylococcus epidermidis; M. sciuri, Mammaliicoccus sciuri.
All 47 isolates were susceptible to rifampin, gentamicin and SYN (Table 4). Multidrug resistance was observed in 17 staphylococcal isolates (36.2%), which showed resistance to ≥3 different antimicrobials agent classes. As shown in Table 4, 41/47 (87.2%) isolates were resistant to FA, displaying the highest frequency of resistance for FA. Among the 47 isolates, 9/10 (90%) S. epidermidis strains and all 32 M. sciuri strains were FA-resistant. However, all the FA resistant S. epidermidis and M. sciuri strains displayed FA MICs ranging from 6–24 μg/mL, indicating low-level resistance (Fernandes, 2016).
MRSA, methicillin-resistant S. aureus; MSSA, methicillin-susceptible S. aureus; OXA, oxacillin; AMP, ampicillin; FOX, cefoxitin; PEN, penicillin; CHL, chloramphenicol; CIP, ciprofloxacin; CLI, clindamycin; ERY, erythromycin; FA, fusidic acid; MUP, mupirocin; SXT, trimethoprim/ sulfamethoxazole; TET, tetracycline; RIF, rifampicin; GEN, gentamycin; SYN, quinupristin/dalfopristin.
All S. epidermidis and M. sciuri isolates showing resistance phenotype to FA were examined for the presence of fusB, fusC, fusD, and fusF. All nine FA-resistant S. epidermidis strains were fusB positive, with one carrying the fusC gene (Table 3). Similarly, all 32 M. sciuri isolates were positive for a fusB-family homolog, fusB/C. None of the FA resistant isolates were carrying fusD nor fusF.
Besides the fusB-family genes, point mutations within the fusA open reading frame encoding EF-G have been associated with FA resistance (Turnidge and Collignon, 1999). As shown in Table 3, a V599I mutation was confirmed in the fusA in only one of the S. epidermidis (SSE9) isolates. No mutation was identified in the fusA gene of M. sciuri isolates from the sequencing analyses.
Discussion
High prevalence of antimicrobial resistance is a significant threat to public health as it undermines treatment options for bacterial infections (Sugden et al., 2016). Since staphylococci are frequently associated with commensal microbiota of skin and mucous surface of various food-producing animals, the development and spread of antimicrobial resistance among staphylococci in the food chain is considered an important threat to food safety (Founou et al., 2016). While coagulase-positive S. aureus has been well investigated for its ability to develop antimicrobial resistance and zoonotic potentials to infect human and animal hosts, relatively few studies have focused on the role of NAS, such as CoNS, in antimicrobial resistance development and transmission. Indeed, several recent studies demonstrated that the antimicrobial resistance in CoNS has been increasing over the past decades (Piette and Verschraegen, 2009), and they act as reservoir for resistance genes that can be transferred to other bacteria (von Wintersdorff et al., 2016).
In this study, we assessed prevalence of staphylococci in retail pork meat and slaughterhouse carcass samples collected from eight provinces of Korea. Overall, the prevalence of staphylococci in the retail pork and slaughterhouse carcasses was 1% and 16.5%, respectively. As shown in Table 2, only 4/44 (9.1%) staphylococci from slaughterhouse carcass samples were S. aureus, and this high proportion of NAS over S. aureus was similar to previous report (Fijałkowski et al., 2016). Among the three different species of NAS from slaughterhouses, M. sciuri displayed the highest prevalence (65.9%) followed by S. epidermidis (22.7%). While previous studies reported much higher levels of S. aureus prevalence in retail pork meat samples in China (18.6%; Wu et al., 2018), Denmark (60%; Tang et al., 2017), and USA (16%–66%; Hanson et al., 2011; O’Brien et al., 2012), no S. aureus was detected from retail pork meat samples in this study. At least several factors such as sample treatment, enrichment/isolation method, and geographical location may have affected the differences in prevalence of S. aureus and other staphylococci. It should also be noted that the use of different sampling methodology (swab samples on ~100 cm2 surface versus 25 g of pork meats) to isolate staphylococci from slaughterhouse carcass samples and retail pork meat samples would have affected the overall prevalence and proportion of each species presented in this investigation.
The occurrence and prevalence of antimicrobial-resistant NAS in retail pork and slaughterhouse carcass samples have not been well investigated in Korea. Recent reports of methicillin-resistant CoNS from food-producing animals have raised concerns regarding transmission of these antimicrobial-resistant staphylococci through the meat production chain (Huber et al., 2011; Nemeghaire et al., 2014b). In this study, 7/44 (15.9%) staphylococci from slaughterhouse carcass samples were methicillin-resistant staphylococci (two MRSA and five MRSE strains) (Table 2). Out of the two MRSA strains, only one strain of S. aureus (SSA1) was ST398 carrying SCCmec V, which has been frequently reported S. aureus genotype in pigs and pork meat worldwide (Chuang and Huang, 2015; Golding et al., 2010; Lozano et al., 2009). Consistent with previous reports (Garza-González et al., 2010; Ruppé et al., 2009), 4/5 MRSE carried SCCmec IV for methicillin resistance. As presented in Table 3, all the M. sciuri isolates displayed a low-level of the OXA resistance phenotype (0.5–2 μg/mL). However, all of these OXA-resistant isolates were susceptible to FOX (Tables 3 and 4), and none of them were positive for the mecA gene. Previously, it has been reported that CoNS isolates other than S. epidermidis strains that displays OXA MICs of 0.5–2 μg/mL may lack mecA (Feßler et al., 2010), and have been defined as methicillin-susceptible strains (CLSI, 2015). It has been suggested that the mecA–negative OXA-resistant CoNS may overexpress penicillinase (Kolbert et al., 1995).
For the last ten years, over a two-fold increase (from 15% to 34%) in FA resistance in clinical isolates of S. aureus has been reported in Korea (Hong et al., 2016). More recently, it has been reported that ~27% of S. pseudintermedius isolates from canine pyoderma and otitis were resistant to FA (Lim et al., 2020). In the current study, as shown in Table 4, 9/10 S. epidermidis strains and the 32 M. sciuri strains displayed FA resistance. Similarly, the high rates of resistance to FA in S. saprophyticus, S. xylosus, and M. sciuri isolates collected from ready-to-eat foods have been reported in Taiwan (Wang et al., 2019). CoNS isolated from meat products also displayed FA resistance rates of 79.2% and 43% in Nigeria (Okoli et al., 2018) and Poland (Fijałkowski et al., 2016) respectively. Recent studies from Taiwan and the UK reported 14% and 46% prevalence, respectively, of FA resistance in clinical isolates of S. epidermidis (Chen et al., 2011; McLaws et al., 2008). The widespread occurrence of FA resistance in non-aureus staphylococcal isolates indicate that NAS such as S. epidermidis and M. sciuri could become a significant public health concern, serving as a reservoir of antimicrobial resistance through food chains. In line with previous reports (Chen et al., 2011; Lee et al., 2018; McLaws et al., 2008), FA resistance in S. epidermidis isolates from slaughterhouse carcasses in this study was mediated by the fusB gene (Table 3). Although one strain of S. epidermidis (SSE3) was double positive for fusB and fusC genes, this strain showed a FA MIC of 8 μg/mL, indicating that the presence of the two fusB-family genes does not confer a high-level FA resistance phenotype (≥ MIC of 128 μg/mL). None of the S. epidermidis isolates displaying FA resistance were positive for fusD nor fusF (Table 3). Sequencing analyses of fusA in S. epidermidis revealed that the SSE9 strain had V599I mutation within the linker site between domain IV and V of EF-G. Amino acid sequence substitutions in EF-G have frequently been associated with high-levels of FA resistance (Fernandes, 2016). However, the location of V599I mutation within EF-G and the relatively low-level FA resistance (MIC of 24 μg/mL) indicate that the point mutation in V599I is not causing FA resistance in the S. epidermidis strain.
The high prevalence of FA resistance in M. sciuri isolated from ready-to-eat-foods (99%) (Wang et al., 2019), healthy chickens (100%) (Nemeghaire et al., 2014b), and livestock (100%) (Bagcigil et al., 2007) has recently been reported. Similar to these reports, all of the M. sciuri isolates from retail pork (n=29) and slaughterhouse carcasses (n=3) exhibited FA resistance (Tables 3 and 4). To determine genetic factors involved in the FA resistance in M. sciuri isolates, a specific primer set detecting homologues of fusB-family genes (fusB/C) was designed in the current study using the published sequences of seven M. sciuri strains in NCBI databases. All the M. sciuri isolates carried the fusB/C gene for the FA resistance phenotype (Table 3), and the sequencing analyses of the amplified PCR products confirmed the sequences of fusB/C genes (data not shown). As shown in Table 5 and Fig. 2, FusB/C protein from M. sciuri displayed 42%–47% of similarity to amino acid sequences of previously characterized FusB-family proteins. None of the 32 M. sciuri strains had point mutation in the fusA gene, correlating with the low-level FA resistance phenotypes observed in the M. sciuri strains. These results combined with the MLST analyses suggests that various genetic lineages of S. epidermidis and M. sciuri strains contribute to the high prevalence of FA resistance in NAS isolated from retail pork and slaughterhouse carcass samples in Korea.
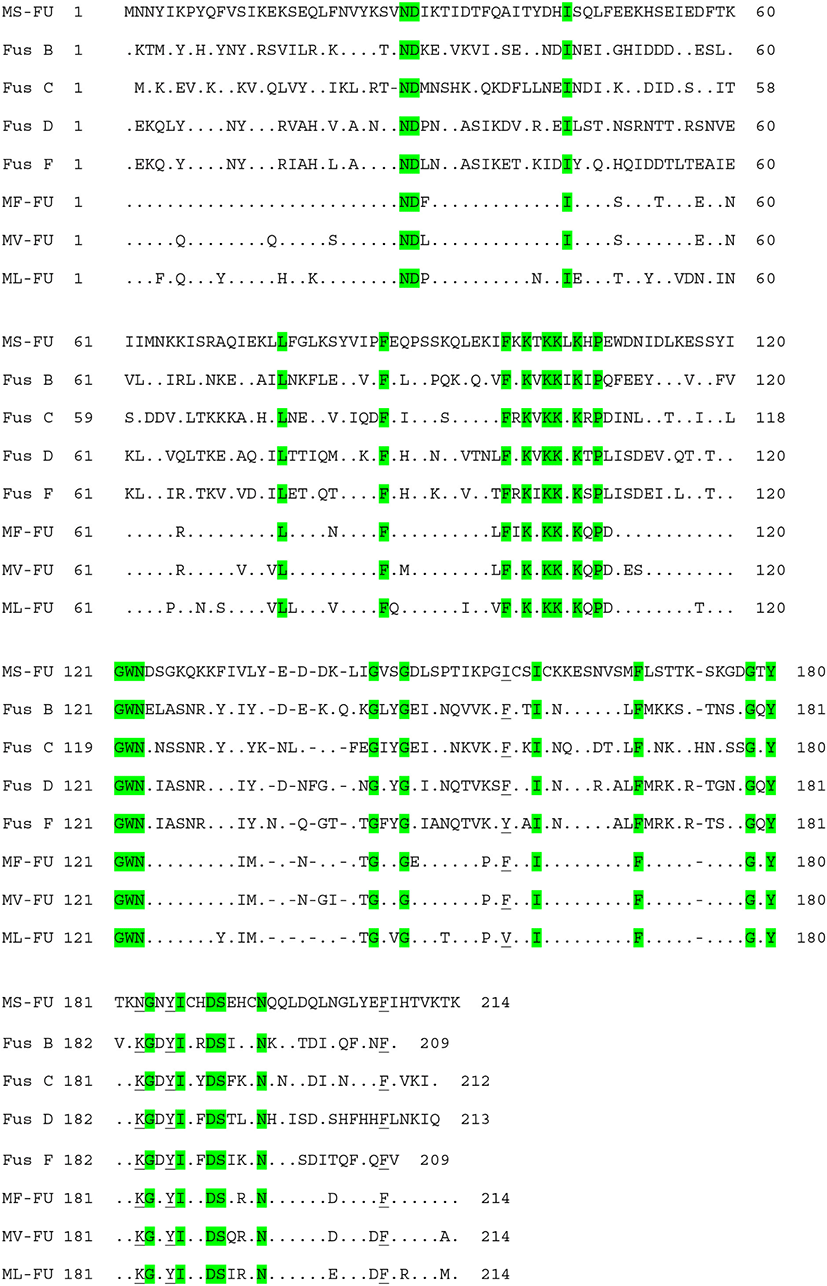
In summary, our results suggest that i) a relatively higher level of NAS than S. aureus are present in pork production chains, particularly in slaughterhouse carcass samples, ii) there is a high prevalence of FA resistance in NAS isolates, especially in S. epidermidis and M. sciuri isolates, and iii) fusB-family genes, rather than fusA mutations, caused the high occurrence of FA-resistant S. epidermidis and M. sciuri. Our results demonstrate a high prevalence of FA-resistant NAS in pork meat production chains, which may act as reservoirs for FA resistance. To the best of our knowledge, this is the first study to report the genetic determinants and prevalence of FA resistance in NAS collected from pork meat production chains in Korea.