Introduction
The global production of vegetable and animal oils has experienced rapid growth due to increasing demand from the food industry and a rising need for non-food applications such as fuel and oleochemicals (Fry and Fitton, 2010; Halloran et al., 2018). Plant cultivation significantly contributes to land use, greenhouse gas emissions, and water consumption, as well as posing a threat to biodiversity (Mungkung et al., 2013). Livestock production trended to face growing impacts from carbon restrictions and environmental and animal welfare (Thornton, 2010). Expanding livestock production to meet consumer needs requires additional land and water resources. Recently, edible insects have emerged as an alternative food source for consumers because of their significant nutritional value, particularly in terms of proteins and lipids. Notably, insect farming requires lower amounts of land, water, and other resources, resulting in reduced environmental impacts and lower emissions of greenhouse gases, such as carbon dioxide, than plant or animal production (Salomone et al., 2017). Oonincx et al. (2010) reported that the emissions of carbon dioxide, methane and nitrous oxide resulting from the farming of edible insects have been found to be lower by a factor of 100 per kg of weight in comparison with Livestock (cattle or pigs). In addition, ammonia emissions of edible insects compare favorably to pigs, with a tenfold difference.
Furthermore, edible insects have the advantages of rapid growth and high bioconversion abilities (Pinotti et al., 2019). Numerous researchers have studied the extraction of alternative proteins from edible insects (Altomare et al., 2020; Choi et al., 2017; Mintah et al., 2020). Certain insects have a higher level of fat than protein, particularly silkworm (Bombyx mori) pupae (SP), sago palm weevil (Rhynchophorus ferrugineus) larvae (PW), and bamboo caterpillars (Omphisa fuscidentalis; BC). Those insects are normally consumed and traded in accordance with the law in Thailand. SP and BC are commonly eaten, whereas PW are well-known edible insects in southern Thailand (Durst et al., 2010). Rumpold and Schlüter (2013) reported that SP (48.7% dry basis) had a lower protein content than crickets (61.2% dry basis) and grasshoppers (62.5% dry basis), but had a higher fat content (30.1% dry basis). The fat and protein contents of BC are 20.4 g/100 g and 9.2 g/100 g, respectively (Durst et al., 2010). PW are rich in lipids, proteins, minerals, and vitamins, with a fat content (52.4%–60.1% dry weight) higher than its protein content (18.0%–28.5% dry weight). They contain chitin in the range of 3.8%–4.5% dry weight (Chinarak et al., 2020). Melo-Ruíz et al. (2016) found that giant water bug (Lethocerus sp.) consisted of fat and protein contents at 8.15 g/100 g (dry sample) and 53.11 g/100 g (dry sample), respectively. In addition, Ghosh et al. (2017) reported the fat contents of five commonly consumed insect species in Korea. They reported that Allomyrina dichotoma larvae, Protaetia brevitarsis larvae, Tenebrio molitor larvae, Teleogryllus emma adult, and Gryllus bimaculatus adult contained fat at 20.24% (DM), 15.36% (DM), 34.54% (DM), 25.14% (DM), and 11.88% (DM), respectively. Kotake-Nara et al. (2002) and Liu et al. (2015) found that SP oil is rich in α-linolenic acid (ALA), an essential fatty acid for the human diet. Furthermore, SP contain an α-glucosidase inhibitor, 1-deoxynojirimycin (DNJ), which might reduce postprandial hyperglycemia and the absorption of carbohydrates (Tomotake et al., 2010). Several researchers have investigated the physicochemical properties of oil extracted from edible insects (Fontaneto et al., 2011; Pino Moreno and Ganguly, 2016; Rossi et al., 2022; Yang et al., 2006); however, little information is available on the physicochemical properties of edible insect oils compared to commonly consumed animal and plant oils.
Therefore, this study investigated the physicochemical properties of oil extracted from edible insects (SP, PW, and BC) and compared them with commonly consumed animal and plant oils [chicken skin (CK), beef back fat (BF), pork back fat (PF), salmon belly (SB), sea bass belly (BB), coconut (C), and peanut (P)]. The chemical compositions of the three edible insects were analyzed. The colors of whole (exoskeleton) edible insects and their extracted oils were evaluated. Edible insect oils were also analyzed for fatty acid profiles and thermal behaviors (melting and crystallization profiles).
Materials and Methods
SP, PW, BC, CK, BF, PF, SB, BB, C, and P were obtained from a supermarket and fresh market in Nakhon Si Thammarat, Thailand. Before physicochemical property analyses and oil extraction, all frozen samples were stored at 4±2°C until the core temperature of the samples were 0°C–4°C.
Oils extracted from all the samples (Bligh and Dyer, 1959) were examined for their fatty acid profiles using gas chromatography (GC) and thermal behaviors (melting and crystallization profiles) using differential scanning calorimetry (DSC).
Oil was extracted from all the samples using the method described by Bligh and Dyer (1959) and modified by Iverson et al. (2001). Briefly, a 25 g sample was first homogenized with a 150 mL mixture of chloroform: methanol: distilled water (1:2:0.9 v:v:v) at 8,991×g for 5 min at 4°C using an IKA homogenizer model T25 D (IKA-Werke, Staufen, Germany). The homogenate was centrifuged at 3,000×g at 4°C for 15 min using a centrifuge (Allegra 25-R, Beckman Instruments, Palo Alto, CA, USA), in which only the chloroform phase was collected. The solvent was evaporated at 30°C using an Eyela rotary evaporator (Tokyo Rikakikai, Tokyo, Japan). All the samples were placed in a plastic container and stored at –20°C until use.
The chemical compositions of all the edible insects were determined. For moisture content, the sample was dried in an oven set to 105±2°C for five hours in order to determine the moisture percentage. After being desiccated and allowed to cool, the dry sample was weighed again. Repetition of the procedure was required to get a constant weight. The Kjeldahl method was used to determine crude protein, and the nitrogen-to-protein conversion factor of 6.25 was multiplied by the total amount of nitrogen to determine total protein content. Fat percentage was calculated by drying fats after extraction in a Soxhlet using Diethyl ether. Ash percentage was calculated by combusting the samples in a silica crucible placed in a muffle furnace. All parameters were analyzed using the AOAC methods (AOAC, 2019). The analytical method numbers used were No. 925.45, 981.10, 948.15, and 923.03, respectively. The carbohydrate content was calculated as the difference between 100 and the sum of the moisture, protein, fat, and ash contents (Mishra et al., 2003).
The color of the whole (exoskeleton) edible insects were measured using a HunterLab colorimeter (ColorQuest XE, HunterLab, Reston, VA, USA) with a 1-inch port size, 10° observers, and illuminant D65. Briefly, samples were placed in a cuvette, and the CIE L*, CIE a*, and CIE b* values were recorded using the CIE color system (Karnjanapratum et al., 2022). The colors of the extracted oils were evaluated using a HunterLab colorimeter (Vista Operation, Hunter Lab) and presented using the CIE color system (CIE L*, CIE a*, and CIE b* values).
Methyl esters of the extracted oil from all the samples were prepared according to the AOAC 991.39 and 969.33 methods (AOAC, 2023). The prepared fatty acid methyl esters were injected into the GC equipped with a flame ionization detector to determine the fatty acid profiles of oils extracted from edible insects, plants, and animals. The Agilent DB-23 column (30 m, 0.25 mm diameter, 0.15 μm film thickness) with helium as the carrier gas was employed for the analysis. Column temperature program began at 50°C for 1 min, then increased 5°C/min to 175°C, and then increased 8°C/min until 225°C. Peaks were identified according to the retention time of standards and the results were expressed as g/100 g of lipid.
The melting and crystallization profiles of the extracted oils were analyzed using DSC (DSC3+, Mettler-Toledo, Schwerzenbach, Switzerland). A liquid nitrogen 20.0 mL/min was used to decrease the oil temperature. Indium was used for both the temperature calibration and the heat flow calibration. A sapphire was used to calibrate the heat capacity within the temperature range of –50.0°C to 100.0°C. Using helium at 25.0 mL/min, the purge gas was administered in accordance with the instrument guidelines. An empty aluminum pan that was hermetically sealed served as the reference sample. The extracted oils (3–6 mg) were weighed in aluminum pans. First, oil samples were cooled from 25°C to –50°C at 5°C/min for crystallization, then heated from –50°C to 100°C at 5°C/min to determine the melting profile (Long et al., 2015). Each crystallization and melting curve was used to determine the T (°C) and ΔH (J/g) values, where Tc-max and Tm-max represent the maximum temperatures in the crystallization and melting curves, respectively. ΔHc-max and ΔHm-max refer to the highest enthalpies in the crystallization and melting curves, respectively.
A completely randomized design was used for the physicochemical properties of all edible insects, using SPSS software (IBM SPSS Statistics, IBM, Armonk, NY, USA). Duncan’s multiple range test was applied for calculating a significant difference among means within each experiment at a significance level of p=0.05.
Results and Discussion
The chemical compositions and colors of edible insects, including SP, PW, and BC, are shown in Table 1. The results revealed that SP (71.66%) had the highest moisture content (p<0.05), followed by BC (68.02%) and PW (67.22%). The fat content of the insects exhibited an inverse relationship with moisture content. PW (20.20%) had the highest fat content, followed by BC (18.18%) and SP (9.69%). The fat content of edible insects is influenced by various factors including species, developmental stage, diet, and environmental conditions (Benzertiha et al., 2020; Meyer-Rochow et al., 2021). Lipids derived from insects have served as an energy source for animal diets, and animal nutrition has effectively integrated the use of insect oils as substitutes for traditional energy sources (Benzertiha et al., 2020). Recently, several studies have investigated the nutritional value of oils extracted from edible insects for human consumption (Banjo et al., 2006; Kotake-Nara et al., 2002; Liu et al., 2015; Tomotake et al., 2010). Banjo et al. (2006) reported 14 species of edible insects in southwestern Nigeria, with fat contents ranging from 1.15% to 31.40%. Kotake-Nara et al. (2002) and Liu et al. (2015) found that the oil extracted from SP contained a high concentration of ALA, which is an essential fatty acid for human nutrition. In addition, they contain a substance called DNJ, which is an α-glucosidase inhibitor that can reduce postprandial hyperglycemia and carbohydrate absorption (Tomotake et al., 2010).
Proteins are important nutrients in edible insects, and SP exhibited the highest protein content (15.79%; Table 1). Higher protein content was also observed in PW (9.30%) than in BC (8.26%); however, PW and BC contained higher levels of fat than protein. Although SP had a lower fat content than protein in the current study, a previous study reported that it (48.7% dry basis) had a lower protein content than crickets (61.2% dry weight) and grasshoppers (62.5% dry weight; Rumpold and Schlüter, 2013). The findings of this experiment revealed that the protein content of three edible insects was inversely proportional to their lipid content.
Carbohydrates were the chemical components observed in the edible insects in the current study. The highest carbohydrate content was found in BC (5.08%), while PW (2.44%) showed a higher carbohydrate content than SP (1.59%). According to Mishra et al. (2003), the carbohydrate content of SP ranged from 1.2% to 1.8%, depending on the silkworm species. Kim et al. (2019) reported that chitin and glycogen are the primary carbohydrates in insects. Chitin, a non-soluble polysaccharide, is present in the exoskeleton of SP (Kim et al., 2016), and PW larvae contain chitin in the range of 3.8%–4.5% (dry weight; Chinarak et al., 2020).
The ash contents of SP, PW, and BC were 1.26±0.00%, 0.83±0.11%, and 0.46±0.01%, respectively. This result was in accordance with previous studies (Chinarak et al., 2020; Mishra et al., 2003). Mishra et al. (2003) found that the ash content of SP was 0.8%–1.4%. The ash content of edible insects varies depending on the species and substances present in their feed (Chinarak et al., 2021). Chinarak et al. (2020) also reported that ash content is related to the level of minerals in PW larvae.
The color values of whole insects (exoskeletons) and oils extracted from SP, PW, and BC are presented in Table 2. Characteristics of three edible insects and color of their extracted oils are shown in Figs. 1 and 2, respectively. SP oil exhibited a significantly lower CIE L* value compared to the other oils, whereas its CIE a* and CIE b* values were the highest (p<0.05). Conversely, the highest CIE L* and lowest CIE b* values were observed in the oil extracted from BC (p<0.05). Thus, the oil extracted from SP had a darker yellow shade than the other samples, which corresponded to the color of the extracted oil shown in Fig. 2. This was probably due to the most intense color of the SP exoskeleton, as indicated by the lowest CIE L* value (27.56) of the whole SP. However, the highest CIE a* and CIE b* values were observed for the whole PW, which was related to the color of all the insects, as shown in Fig. 1. The pigment in SP was identified as a carotenoid. Generally, animals cannot synthesize carotenoids themselves and must obtain them from their diet. SP acquire carotenoids from mulberry leaves (Chieco et al., 2019) because they contain abundant carotenoids, particularly lutein and β-carotene. In addition, their leaves contain other carotenoids such as antheraxanthin, violaxanthin, and neoxanthin (Chieco et al., 2019). The extracted oil from SP had a more yellow-red hue compared to palm oils, while PW and BC had a color similar to that of palm oils, with CIE L*, CIE a*, and CIE b* values of 96.4 to 97.74, –6.05 to –6.62, and 28.11 to 33.33, respectively (Rossi et al., 2001).

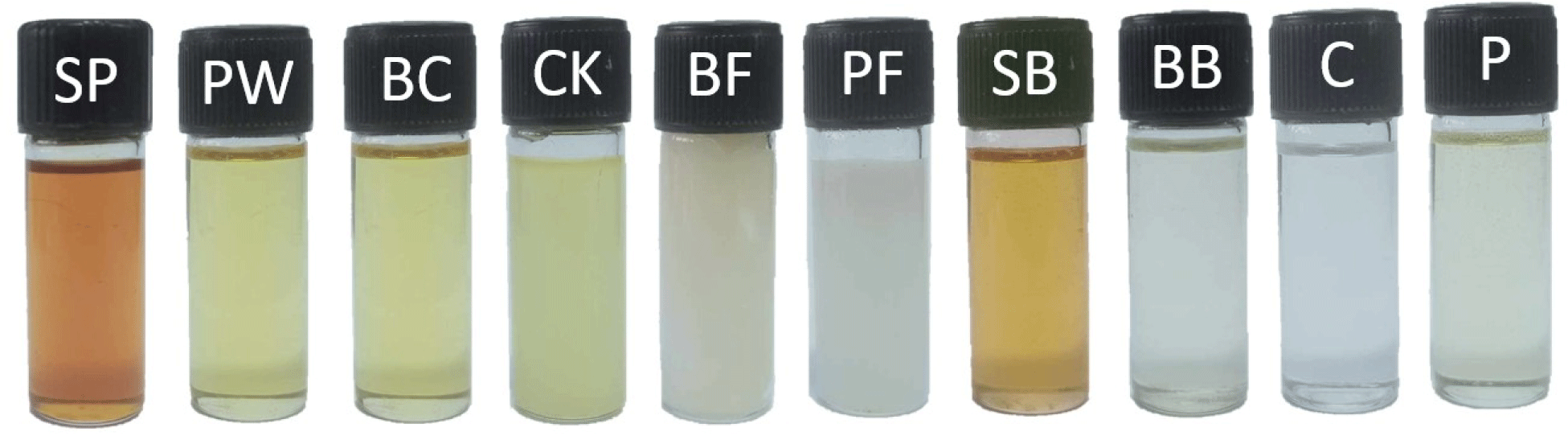
The fatty acid profiles of oil extracted from the three edible insects are shown in Tables 3, 4, and 5. Saturated fatty acids (SFAs), monounsaturated fatty acids (MUFAs), and polyunsaturated fatty acids (PUFAs) were reported.
The SFA contents of the extracted oil from PW and BC were higher than that from CK, PF, SB, BB, and P oils. SP oil had SFA levels in the same range as that of CK and BB oils (Table 3), and the oil extracted from SB had the lowest SFA content. Several countries advise that high SFA consumption be avoided and to replace saturated fats with cis-unsaturated fats (Brouwer, 2020). According to dietary guidelines, the recommended daily intake of saturated fat is <7%–10% of the daily energy because SFA increases low-density lipoprotein (LDL) and total blood cholesterol levels, which increases the risk of coronary heart disease (Parodi, 2016). Herein, lauric (C12:0), myristic (C14:0), palmitic (C16:0), stearic (C18:0), arachidic (C20:0), and behenic acids (C22:0) were detected in all the extracted oils (Table 4). Palmitic acid (C16:0) was more abundant in all extracted oils than the other fatty acids. The fat extracted from BC contained the highest quantity of palmitic acid (C16:0), followed by that from SP. Lauric acid (C12:0) in all the edible insect oils was in the same range as that in all extracted oils, except for C. Compared to all other edible insect oils, the oil from C had a higher level of SFA, particularly lauric acids (C12:0), myristic acids (C14:0), and palmitic acids (C16:0), which were more abundant in C oil. In a previous study, all the SFAs equally contributed to an increase in cholesterol levels caused by animal-based fats. Rent evidence indicated that the primary contributors to the elevated levels of total and LDL cholesterol in the bloodstream are lauric, myristic, and palmitic fatty acids (Williams, 2000). This information revealed that BC was an oil with a worse fatty acid quality than SP and PW due to its high palmitic acids (C16:0) content. Conversely, stearic acid, another significant SFA, has no effect on total or LDL cholesterol levels (Williams, 2000). Notably, SP oil had a higher level of stearic acid than the PW and BC oils.
The MUFAs in the oil extracted from edible insects, animals, and plants are presented in Table 3. The MUFA content of the extracted oil from BC was 51.36 g/100 g, which was higher than that of PW (47.65 g/100 g) and SP (36.54 g/100 g). Edible insect oils had a comparable amount of MUFAs to that of all samples (ranging from 36.54 g to 51.36 g/100 g), except for C, which had the lowest MUFA content (4.84 g/100 g). Although the decrease in cholesterol was affected by PUFAs more than by MUFAs, MUFAs are an advantageous fatty acid when substituting SFAs in the diet (Williams, 2000). MUFAs can reduce the level of LDL cholesterol, but not reduce the level of high-density lipoprotein cholesterol. Therefore, MUFAs have a greater potential for use in cholesterol-lowering diets than previously recognized (Grundy, 1989). PW and BC contained higher concentrations of palmitoleic acid (C16:1 n-7) than the other samples (Table 5). Palmitoleic acid, particularly its cis isoform, has received increasing attention because of its potential to enhance insulin sensitivity, reduce lipid accumulation in the liver, and decrease the expression of pro-inflammatory markers and adipokines. These effects are associated with the development of metabolic abnormalities (Frigolet María and Gutiérrez-Aguilar, 2017). Higher concentrations of oleic acid (C18:1 n-9) were found in all the extracted oils than those of the other fatty acids. According to Calder (2015), oleic acid is the most frequently observed acid in foods. Oleic acid can be obtained from various plant oils and animal-derived fats, including lard, tallow, and butter. In the present study, the levels of oleic acid were higher in PW and BC than in SP. Compared to PUFAs, oleic acids provide a significant level of protection against LDL oxidation, thereby reducing the development of proatherogenic oxidized LDL. The reduced peroxidizing ability of oleic acid in lipoproteins and cell membranes, as opposed to PUFAs, likely restricts inflammation because oxidative stress promotes inflammation (Calder, 2015).
The oil extracted from SP contained a higher concentration of PUFAs compared to that from PW, BC, CK, BF, and PF, but was similar in concentration to SB and BB (Table 3). The oil extracted from C had the lowest PUFA content. PUFAs in food are essential to human health, and a previous study proposed that long-chain PUFAs, particularly those belonging to the omega-3 (n-3) series, played a significant role in human evolution by providing critical components for cerebral tissue development (Fontaneto et al., 2011). Linoleic acid (C18:2 n-6) was found in the oil extracted from all the samples and was the only PUFA detected in the oil extracted from C (Table 5). The highest linoleic acid content was observed in SP oil compared to all the edible insect oils in the current study. Additionally, SP oil had the greatest content of ALA (C18:3 n-3) among all the samples. This result was consistent with the reports of Kotake-Nara et al. (2002) and Liu et al. (2015), who determined that the oil derived from SP had a significant amount of ALA. Linoleic acid is the initial fatty acid that results in the production of the bioactive omega-6 PUFA, arachidonic acid (ARA). Moreover, ALA is the initial fatty acid that results in the production of the bioactive omega-3 PUFAs, eicosapentaenoic acid (EPA) and docosahexaenoic acid (DHA; Williams, 2000). Swanson et al. (2012) stated that omega-3 fatty acids are associated with promoting healthy aging throughout the life cycle, and EPA and DHA typically produce anti-inflammatory eicosanoids. Therefore, consuming a greater amount of n-3 PUFAs could provide protection against inflammatory illnesses, cancer, cardiovascular disorders, and other chronic diseases (Saini and Keum, 2018). High concentrations of ARA, EPA, and DHA were present in the extracted fish oils (SB and BB); in contrast, low concentrations of ARA, EPA, and DHA were observed in all edible insect oils. ARA (0.01–0.02 g/100 g) was in all edible insect oils lower than CK, BF, PF, SB, and BB oils. SP oil (0.03 g/100 g) showed a slightly higher level in EPA than PW (0.01 g/100 g) and BC (0.01 g/100 g) oils. DHA was found in SP (0.03 g/100 g) and BC (0.01 g/100 g) oils but could not be detected in PW oil.
Hamidah et al. (2011) compared the fatty acid profiles of palm and soybean oils and reported that palm oil had a higher concentration of SFAs than of MUFAs and PUFAs. The highest concentration of PUFAs compared to SFAs and MUFAs was found in soybean oil. Compared with edible insect oils, oil extracted from edible insects showed the highest concentrations of palmitic and oleic acids, similar to both palm and soybean oils. Palm oil exhibited the highest levels of palmitic (C16:0) and oleic acids (C18:1 n-9), whereas soybean oil had the highest levels of linoleic acid (C18:2 n-6), followed by palmitic (C16:0) and oleic acids (C18:1 n-9). Edible insect oil (35.28–43.70 g/100 g) contained oleic acid (C18:1 n-9) in a similar range to that of palm oil [39.65 g/100 g; Hamidah et al. (2011)] but higher than that of soybean oil [20.98 g/100 g; Hamidah et al. (2011)]. SP oil (24.60 g/100 g) had a higher ALA (C18:3 n-3) content than both palm oil [0.27 g/100 g; Hamidah et al. (2011)] and soybean oil [8.18 g/100 g; Hamidah et al. (2011)].
Although the fat content in SP was lower than that in PW and BC, the fat contents and fatty acid profiles of edible insect oils revealed that the oil extracted from SP contained more essential fatty acids, such as PUFAs, than that in PW and BC. This result revealed that oil extracted from edible insects could be a future alternative fat source. Moreover, PW contained the highest fat content compared to SP and BC, and PW oil also contained a higher concentration of MUFAs than SFAs. Although BC oil had a higher fat level than SP oil, it contained more SFAs than MUFAs and PUFAs. The result of the present study revealed that BC was an oil with a worse quality than SP and PW, as indicated by the higher levels of SFAs and fatty acid profiles.
Crystallization and melting characteristics of all extracted oils in terms of T (°C), ΔH (J/g), and transition peak temperatures of peaks 1 and 2 are presented in Tables 6 and 7, respectively. Tc-max and Tm-max represent the maximum temperatures in the crystallization and melting curves, respectively. ΔHc-max and ΔHm-max refer to the highest enthalpies in the crystallization and melting curves, respectively. The crystallization (Table 6) and melting curves (Table 7) of all the extracted oils displayed two peaks, except C oil, as well as chicken oil for the crystallization curve.
All the edible insect oils had Tc-max values (–15.83°C to –19.08°C) higher than that of CK (–39.92°C), SB (–35.92°C), and BB (–28.08°C; Table 6). In contrast, the Tc-max values of all the edible insect oils were lower than BF (2.83°C), C (4.75°C), and P (–2.92°C). Matthäus et al. (2019) reported that palm kernel oil had a Tc-max of 1.82°C, which was higher than that of extracted oils from all the edible insects in the current study. Srivastava et al. (2017) stated that oils that exhibit low-temperature resistance to crystallization are highly stable fats. The crystallization enthalpy (△Hc-max) value of SP oil (19.51 J/g) was lower than that of PW oil (35.46 J/g) and BC oil (36.73 J/g). The highest △Hc-max value was found in C oil, which was related to the concentration of SFAs (Table 3). The crystallization conditions and complex mixture of triglycerides exhibit a phase similar to that of polymers by changing the molten state of the oil to undergo nucleation, activation, crystal growth, and crystal lattice stages (Srivastava et al., 2017). The crystallization and melting properties are significantly influenced by the composition of fatty acids and triacylglycerols. This affects the physical attributes of fats, such as the mouthfeel and consistency of foods (margarines, shortenings, and confectioneries; Matthäus et al., 2019). Crystallization is also used to determine the properties of fats and oils during storage at low temperatures (Metin and Hartel, 2005). The current study revealed that all the edible insect oils did not easily crystallize at low temperatures, as indicated by the low temperature of crystallization (°C–15°C).
The melting behaviors of all the extracted oils are presented in Table 7. SP (–20.08°C) and P (–20.58°C) oils had lower Tm-max values than the other samples. For edible insect oils, a lower Tm-max value was observed in SP oil than in PW (16.42°C) and BC (16.25°C) oils, which was related to their level of SFAs (Table 3). A high melting point represents SFAs, whereas a low melting point represents UFAs (Phuah et al., 2024). Compared to that for soy bean and palm kernel oils, the Tmax value of soy bean oil (–22.97°C) in the melting curve (Hayati et al., 2009) was lower than that of edible insect oils (SP=–20.08°C, PW=16.42°C, and BC=16.25°C) in the current study, while palm kernel oil (25.23°C) exhibited a higher Tmax value (Matthäus et al., 2019). The findings indicated that all the edible insect oils were in a liquid state at room temperature (25°C), thus resembling other extracted oils, except for the oil recovered from C (Tm-max of 24.75°C). PW and BC oils underwent a phase change from solid to liquid when exposed to temperatures <16°C therefore, they were in the solid stage under cold conditions. The highest melting enthalpy (△Hm-max) values were found in C oil. C oil (114 J/g) exhibited the higher △Hm-max value than edible insect oils (22.09–37.40 J/g). This was probably because of its high concentration of medium-chain SFAs (Birker and Padley, 1987). The crystallization and melting curves of C oil exhibited only one sharp peak because it comprised an extremely high concentration of SFAs up to 94.23 g/100 g and low concentrations of MUFAs and PUFAs. The melting enthalpy (△Hm-max) values of all the edible insect oils were lower than that of all the samples, except SB oil. A minimal amount of energy was required to melt oils extracted from edible insects. This result indicated that a minimal amount of energy was required to melt oils extracted from edible insects, become liquid at room temperature, and minimize oil loss during industrial extraction processes (Tzompa-Sosa et al., 2019).
Conclusion
All the edible insect oils had the same fatty acid profile as that of the commonly consumed animal and plant oils measured in this study, which were abundant in palmitic (C16:0) and oleic acids (C18:1 n-9). SP oil had a lower fat content than PW and BC oils but had more essential fatty acids and PUFAs. The fatty acid profile of SP oil was similar to that of fish oil, although it contained less ARA, EPA, and DHA. PW had the highest fat content compared to SP and BC, and PW oil also had a greater proportion of MUFAs than SFAs. Although BC oil had a higher fat level than SP oil, it contained more SFAs than MUFAs and PUFAs. The crystallization and melting properties of all the edible insect oils were investigated, and all oils were liquid at ambient temperatures and did not crystallize easily at low temperatures. Moreover, the melting of edible insect oils required minimal energy. This study suggests that edible insect oils, particularly SP oil, can serve as alternative oil sources to meet the increasing demand for oil in the future.