Introduction
Meat tenderness is one of the most important indicators used by consumers in evaluating meat quality, along with flavor and juiciness (Jeremiah, 1982). The three factors with the greatest influence on the shear force of meat are the collagen content, shortening, and aging (Bhat, 2018; Jeong et al., 2023; Jo et al., 2024). Various factors such as the ultimate pH, animal species, fat content, and the ratio of muscle fiber types also influence the tenderness of meat during production, and extensive research has sought to identify the interaction between various factors and the correlation with meat tenderness (Kim et al., 2023; Marino et al., 2013).
Aging, the storage of meat under microbiologically safe conditions, is widely used to improve the sensory quality of meat. The main factor influencing the increase in the sensory quality of meat during aging is the breakdown of myofibrillar proteins due to the activity of proteolytic enzymes in the muscles. These enzymes weaken muscle structures and produce protein breakdown products that act as flavor precursors (Lee et al., 2024; Li et al., 2022; Toldrá and Flores, 1998). Additionally, reports indicate that meat aging enhances the in vitro protein digestibility of meat (Lee et al., 2023a). Proteolytic enzymes in muscle include calpain, cathepsin, caspase, and proteasome, the activity of which is known to be affected by external factors such as the aging conditions and internal factors such as the biochemical and genetic properties of the muscle (Ertbjerg, 2022). Among these endogenous proteases, calpains and cathepsins are the most studied in relation to meat tenderness (Jiang, 2000). These proteolytic enzymes break down structural proteins and weaken the structural stability of muscle fibers, contributing to improved tenderness (Lonergan et al., 2010; Taylor et al., 1995). Studies have shown that the shear force, a key indicator of meat tenderness, is influenced by the breakdown of muscle proteins during aging, a process known as proteolysis (Marino et al., 2013). A decrease in the shear force, indicating an improvement in meat tenderness due to postmortem protein degradation, has been reported in previous studies (Phelps et al., 2016; Schulte et al., 2019).
From industrial and research perspectives, aging is more commonly used to improve beef tenderness. Based on long-term research, the effect of improving meat quality through aging appears to be clear; however, unlike beef, the effect of improving tenderness through aging in pork is controversial (Hwang et al., 2018; Koohmaraie et al., 1991; Setyabrata et al., 2021). Physicochemical properties, such as protease activity, muscle fiber type, pH, collagen content, and sarcomere length, may differ for pork and beef (Bhat, 2018). Thus, we expected that variations in the intrinsic properties of the two species would cause differences in the tenderness of pork and beef. Although individual studies have explored these parameters in specific species (Holman et al., 2020; Wheeler et al., 2000), a comparative study should be conducted.
Thus, the primary objective of this study is to determine the differences in the proteolytic behavior and shear force during the aging of pork and beef. The electrical conductivity, along with the activities of calpain and cathepsin B, the key proteases involved in meat protein degradation, are monitored over 14 days of aging of both pork and beef semitendinosus muscles. To focus on the proteolytic patterns and shear force, semitendinosus muscles with low-fat content and regular orientation of the muscle fibers were used herein in both species. Additionally, the shear force of the cooked meat during aging is assessed to understand the alterations in the tenderness of these muscle types. By examining these parameters during aging, we aim to identify the different patterns that contribute to the overall tenderness of pork and beef.
Materials and Methods
Semitendinosus muscles of beef (Hanwoo) and pork [(Landrace×Yorkshire)×Duroc] were purchased in the local market and used for aging. The muscles were sourced from three distinct pork and beef carcasses collected 24 and 48 h postmortem, respectively. In the case of beef, a single muscle was obtained from each carcass, whereas two muscles (left and right) were acquired from pork because of the shorter muscle length compared to that of beef. The appearance, meat color, and pH of the beef and pork semitendinosus muscles are presented in Supplementary Fig. S1 and Supplementary Tables S1 and S2. The muscles were cut to a length of 5 cm and vacuum-packed. Subsequently, two muscles were randomly assigned to each storage day; thus, six muscles per storage day (0, 7, and 14 days) were used in the experiment (three batches from three carcasses, each with two replicates). For the aging process, the vacuum-packed muscles were stored at 4°C for 7 or 14 days. At the end of the aging period, half of the samples were ground (FPM250, Kenwood, Havant, UK) and used for physicochemical analysis. For the shear force analysis, the other half of the sample was vacuum-packed again and cooked at 80°C until the core temperature reached 75°C in a water bath. The samples for the shear force tests were cut into 5 cm (length)×1.3 cm (diameter) pieces. The shear force was measured using an A-XT2 texture analyzer (Stable Micro Systems, Surrey, UK). The speed was set to 2.0 mm/sec, and the measured cutting depth was 4 cm.
The electrical conductivity was measured at 200 different points ranging from 40 Hz to 200 kHz using an LCR meter (IM3533-01, Hioki, Nagano, Japan). The electrode used in the LCR meter was a 10×10 mm bar with a separation distance of 10 mm between the two electrodes. The electrical conductivity was assessed three times by placing the sample in the holder parallel to the direction of the muscle fiber.
Cathepsin B activity was measured with a cathepsin B substrate (Z-Arg-Arg-AMC, Sigma-Aldrich, St. Louis, MO, USA). An aliquot (8 mL) of the extraction buffer (10 mM KH2PO4, 1 mM EDTA, 50 mM NaCl, and 250 mM sucrose; pH 7.4) was added to 2 g of the meat sample, homogenized at 13,000 rpm for 30 s (T25 basic, IKA-Werke, Staufen, Germany), and centrifuged at 3,000×g for 10 min (1580R, LABOGENE, Lynge, Denmark). The supernatant was filtered through a 1.0 mm stainless steel mesh to remove the connective tissue and centrifuged again at 3,000×g for 10 min. The supernatant was diluted to a protein content of 0.25 mg/mL. Afterward, 15 μL of the sample, 135 μL of reaction buffer (4 mM EDTA, 60 mM acetic acid, 340 mM sodium acetate, and 0.1% Brij 35; pH 5.0), and 100 μL of 12.5 μM cathepsin B substrate in methanol were added and the mixture was reacted for 10 min at 40°C. The fluorescence of the samples was measured using a plate reader (Varioskan LUX, Thermo Fisher Scientific, Waltham, MA, USA) at an excitation wavelength of 355 nm and an emission wavelength of 460 nm.
The calpain activity was monitored using a calpain substrate (Suc-Leu-Leu-Val-Tyr-AMC; Abcam). The samples were extracted by adding 4 mL of PBS (2.7 mM KCl, 137 mM NaCl, 10 mM NaHPO4, and 1.8 mM KH2PO4; pH 7.4) to 2 g of the meat sample, which was then homogenized twice by mixing for 30 s at 13,000 rpm and centrifuged at 3,000×g for 10 min (1580R). The supernatant was filtered through a 1.0 mm stainless steel wire mesh to remove the connective tissue, centrifuged again at 3,000×g for 10 min (1580R), and diluted to a protein content of 0.25 mg/mL. An aliquot (85 μL) of the extracted sample, 10 μL of 10X reaction buffer, and 5 μL of 40 μM calpain substrate were mixed and reacted at 37°C for 60 min. After the reaction was complete, the fluorescence of the mixture was measured at an excitation and emission wavelengths of 400 and 505 nm, respectively (Varioksan LUX).
The activities of calpain and cathepsin B are expressed as n-fold increases relative to those observed in the meat on Day 0.
The content of α-amino groups in the 10% trichloroacetic acid (TCA)-soluble fraction was determined following the method of Lee et al. (2024) using o-phthaldialdehyde reagent. A standard curve was prepared using glycine; the α-amino group content in the sample is expressed in mM/g. The protein content of the samples was measured using the Kjeldahl method (AOAC 928.08).
The preparation of myofibrillar protein extract and sodium dodecyl sulfate-polyacrylamide gel electrophoresis (SDS-PAGE) were conducted according to our previously reported method (Lee et al., 2024). After adjusting the protein content of the myofibrillar protein extract with 2 mg/mL, SDS-PAGE was performed using a 12.5% polyacrylamide gel with a protein ladder (3454A, Takara Bio, Shiga, Japan) in a Page Run device (AE-6531 mPAGE, ATTO, Tokyo, Japan).
An aliquot (30 mL) of cold isolation buffer [2 mM MgCl2, 0.1 M KCl, 1 mM ethylene glycol tetraacetic acid (EGTA), and 10 mM potassium phosphate; pH 7.0] was added to 5 g of the sample and homogenized at 12,000 rpm for 15 s (T25 basic). The homogenate was centrifuged at 2,000×g for 10 min (1580R) to remove the supernatant. The pellet was homogenized in 30 mL Tris buffer (5 mM Tris, 1 mM DTT, and 1 mM EGTA; pH 7.8). Connective tissue was removed using a 1.00 mm mesh strainer, and the homogenate was centrifuged at 2,000×g for 10 min (1580R). Thereafter, 30 mL of phosphate buffer (150 mM potassium phosphate and 150 mM EDTA, pH 6.5) was added to the pellet and homogenized at 12,000 rpm for 30 s (T25 basic). The homogenate was stirred at 4°C for 20 min and then centrifuged at 2,000×g for 10 min (1580R) to remove the supernatant. An aliquot (30 mL) of Guba-Straub buffer (0.4 M NaCl, 150 mM Na3PO4, 5 mM MgCl2, and 5 mM Na4P2O7; pH 6.0) was added to the pellet and homogenized at 12,000 rpm for 30 s (T25 basic). The homogenate was again stirred at 4°C for 20 min and centrifuged at 2,000×g for 10 min (1580R). Distilled water was added to the supernatant (1:1, v/v), and the mixture was homogenized and centrifuged at 2,000×g for 10 min (1580R). The final pellet was homogenized with 4 mL of 0.1 M potassium phosphate (pH 7.0) at 12,000 rpm for 30 s (T25 basic) and used as the myosin extract.
The thermal denaturation temperature of the myosin extract (protein concentration of 0.5 mg/mL) was determined by circular dichroism (CD) spectroscopy (Chirascan VX, Applied Photophysics, Leatherhead, UK). The CD data (millidegrees) were obtained by increasing the temperature of the sample chamber from 25°C to 98°C at a heating rate of 5°C/min. The thermal denaturation temperature of the myosin extract was selected as the point at which the slope of the graph changed during the first differentiation.
Three batches of analyses were performed using semitendinosus muscles from three pork and beef carcasses. Statistical analysis was performed using a mixed model with a randomized complete block design in SAS (version 9.3; SAS Institute, Cary, NC, USA). Each replicate was treated as a block. The results are presented as the mean value and SEM. Significance testing for the main effect was conducted at the p<0.05 level using Tukey’s multiple tests.
Results and Discussion
Muscle is composed of several muscle cells surrounded by an insulating cell membrane. The electrical properties of meat change due to changes in the postmortem composition of the intracellular and extracellular fluids that serve as electrolytes (Jo et al., 2023). Electrical conductivity, a measure of the degree to which electricity flows within an object, can be used to assess the degree of damage to the membrane system of postmortem muscle tissues (Byrne et al., 2000). The electrical conductivity was measured in the range of 40 Hz to 200 kHz (Fig. 1). The electrical conductivity of pork increased on Day 7 compared to Day 0, but there was no significant difference between Day 7 and 14. However, the electrical conductivity of beef on Day 14 increased compared to that on Day 0 and 7, but there were no change in the electrical conductivity between Day 0 and 7.
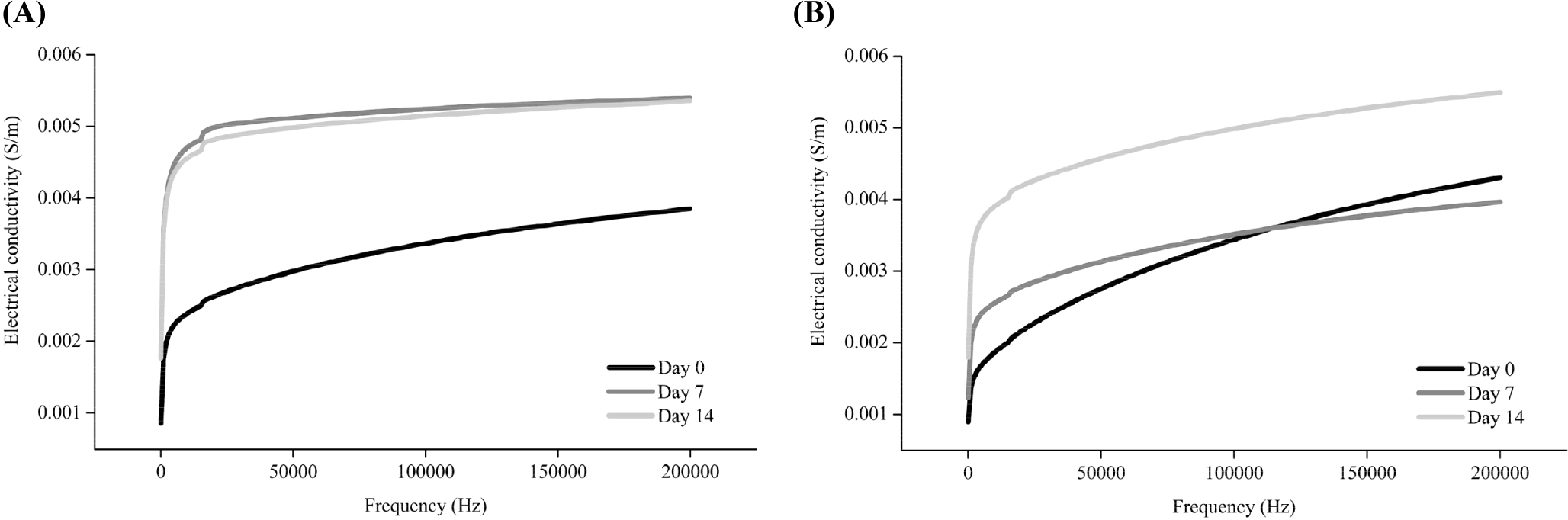
Previous studies have reported an increase in the electrical conductivity of meat following postmortem storage (Callow, 1936; Pliquett et al., 1995; Salé, 1976; Swatland, 1980). As the storage period after the slaughter of animals increases, the cell membrane is weakened due to apoptosis, and large amounts of ions present in cellular organelles are released (Feidt and Brun-Bellut, 1996; Ouali et al., 2006). Although the degradation of connective tissue during postmortem storage of muscles is still controversial (Chizzolini et al., 1977; Nishimura, 2015; Stanton and Light, 1990), structural alteration of connective tissue can also potentially affect the changes in the electrical conductivity. Thus, the increase in the electrical conductivity following aging of pork and beef may have been due to the weakening and loss of integrity of the muscle cell, which can also affect the activity of endogenous proteolytic enzymes.
The aging time at which the electrical conductivity changed differed for the two species. These differences might be attributed to differences in the fiber compositions of the pork and beef semitendinosus muscles. Considering the muscle fiber composition of the semitendinosus muscle, red and white muscle fibers constitute 10%–20% and 64%–80% of pork, respectively (Semenova et al., 2023), whereas beef contains 10%–30% and 34%–58%, respectively (Totland et al., 1988). White muscle fibers are known to have a lower water-holding capacity than red muscle fibers because of their strong glycolytic activity, resulting in the exudation of more water (Kim et al., 2013; Lyu et al., 2024). The purge loss in pork (3.22% and 3.61% higher on Day 7 and 14, respectively) was also higher than that in beef (2.11% and 2.18% on Day 7 and 14, respectively; p<0.05, data not shown). Once intracellular moisture is released along with the ions present within the cells, the electrical conductivity changes (Byrne et al., 2000; Pliquett et al., 1990). Thus, we assumed that the faster and greater loss of intracellular moisture in pork might have induced a faster increase in the electrical conductivity than in beef. This result is consistent with a study by Byrne et al. (2000), who reported a low water-holding capacity and a rapid increase in the electrical conductivity in fast glycolytic muscles. A decline in the pH can also affect weakening of the cell membrane, where Lyu et al. (2024) reported that the abundance of red (oxidative, type I) muscle fibers slows down the pH decline during aging. Consistently, in this study, the pH decreased on Day 7 and 14 in pork and beef, respectively (p<0.05, Supplementary Table S1). Therefore, the timing of changes in the electrical conductivity differed for the two species owing to variations in the timing of intracellular moisture movement and pH changes, which resulted from the differences in the muscle-fiber composition of pork and beef.
Calpain and cathepsin B are two key endogenous proteases for the postmortem protein degradation of meat (Bhat, 2018; Kaur et al., 2021). Calpains and cathepsins are two key enzymes in postmortem aging (Lomiwes et al., 2014). Calpains undergo autolysis upon exposure to sufficient Ca2+ for activation, which affects their proteolytic activity (Bhat et al., 2018). Cathepsin B, typically inactive in lysosomes, may become active in the cytoplasm after slaughter (Ahmed et al., 2015; Lomiwes et al., 2014). The calpain and cathepsin B activities were measured to determine the protease activity of pork and beef according to the storage period (Fig. 2). The calpain activity increased significantly between Day 7 and 14 in pork (p<0.05) and decreased in beef on Day 7 (p<0.05). According to previous research, the absolute calpain content is higher in red muscle fibers. However, the ratio of calpastatin, an inhibitor of calpain activity, to calpain is higher in red muscle fibers; therefore, the calpain activity appears to be higher in white muscle fibers than in red ones (Koohmaraie, 1996). Thus, the higher calpastatin to calpain ratio in beef might have resulted in a decrease in calpain activity unlike pork (Geesink and Koohmaraie, 1999). It appears that the calpain activity differed depending on the main type of muscle fiber comprising pork and beef. In addition, the muscle pH during storage was higher in pork (6.03, 5.90, and 5.91 on Day 0, 7, and 14, respectively) than in beef (5.56, 5.57, and 5.51 on Day 0, 7, and 14, respectively). Because calpain exhibits optimal activity in the neutral pH range (pH 6–7, Kaur et al., 2021), pH might also contribute to the higher calpain activity in pork during aging than in beef.
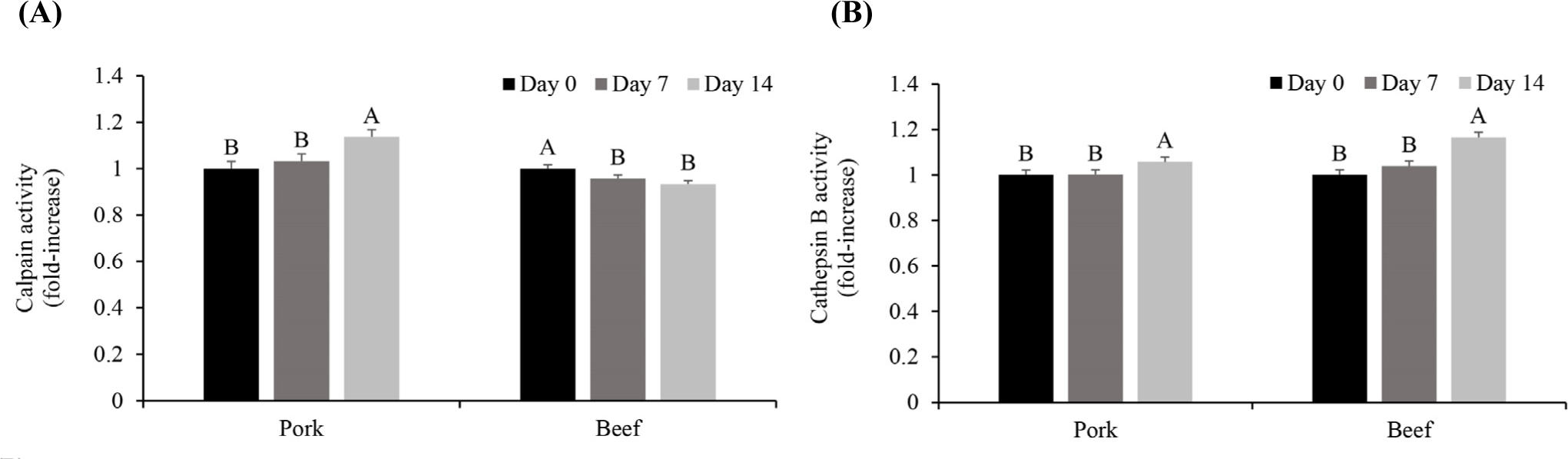
The cathepsin B activity increased significantly between Day 7 and 14 in both pork and beef (p<0.05, Fig. 2). The activity of cathepsin B, which is present in lysosomes, is expressed subsequent to release into the cytoplasm when the lysosomal membrane is weakened owing to a postmortem pH decline (Koohmaraie, 1992). Zeece et al. (1992) reported that most lysosomes are degraded within 14 days postmortem. As previously shown in the electrical conductivity tests during aging, the electrical conductivity in both pork and beef increased as the aging period increased (Fig. 1). Thus, along with disruption of the lysosomal membrane, cathepsin B appears to have been released and exhibited its activity during storage. A higher increase in cathepsin B was observed in beef on Day 14 (approximately 16% increase compared to Day 0) than in pork (approximately 5% increase compared to Day 0). Although the role of cathepsin B in the postmortem proteolysis of meat is controversial (Kaur et al., 2021; Sentandreu et al., 2002), our previous studies have consistently observed the increase in the cathepsin B activity during aging in beef (Lee et al., 2021; Lee et al., 2023b). Because the activity of cathepsin B is optimal in the acidic region near pH 5.5 (Kaur et al., 2021), the lower pH in beef than that in pork could contribute to the greater increase in the cathepsin B activity in the former during aging.
Depending on the aging period, the extent of proteolysis also differed for pork and beef. During meat aging, the content of small peptides and amino acids increases due to cleavage of the peptide bonds by endogenous proteases (Lonergan et al., 2010). Because small peptides with 3-4 residues are solubilized in 10% TCA (Lee et al., 2024), we monitored the α-amino group content in the 10% TCA-soluble fraction of beef and pork (Fig. 3). In pork, there was no significant difference in the α-amino group content on Day 0 and 7 (p>0.05), but a significant increase was observed on Day 14 (p<0.05). In contrast, the α-amino group content in beef increased gradually with prolonged aging (p<0.05). On Day 0, the α-amino group content in pork was 34.94 mM/g, which was significantly higher than that in beef (28.94 mM/g; p<0.05). The pH of white or intermediate muscle fibers decreases more rapidly than that of red fibers in the early post-mortem stage (Shen et al., 2015), which triggers Ca2+ release from the sarcoplasmic reticulum. Calpain-1 is primarily involved in muscle protein degradation after autolysis (Bhat, 2018). Consequently, we assume that proteolysis in pork was initiated on Day 0 by calpain, where the increase in the α-amino group content on Day 14 is attributed to later activation of cathepsin B compared with calpain activation.
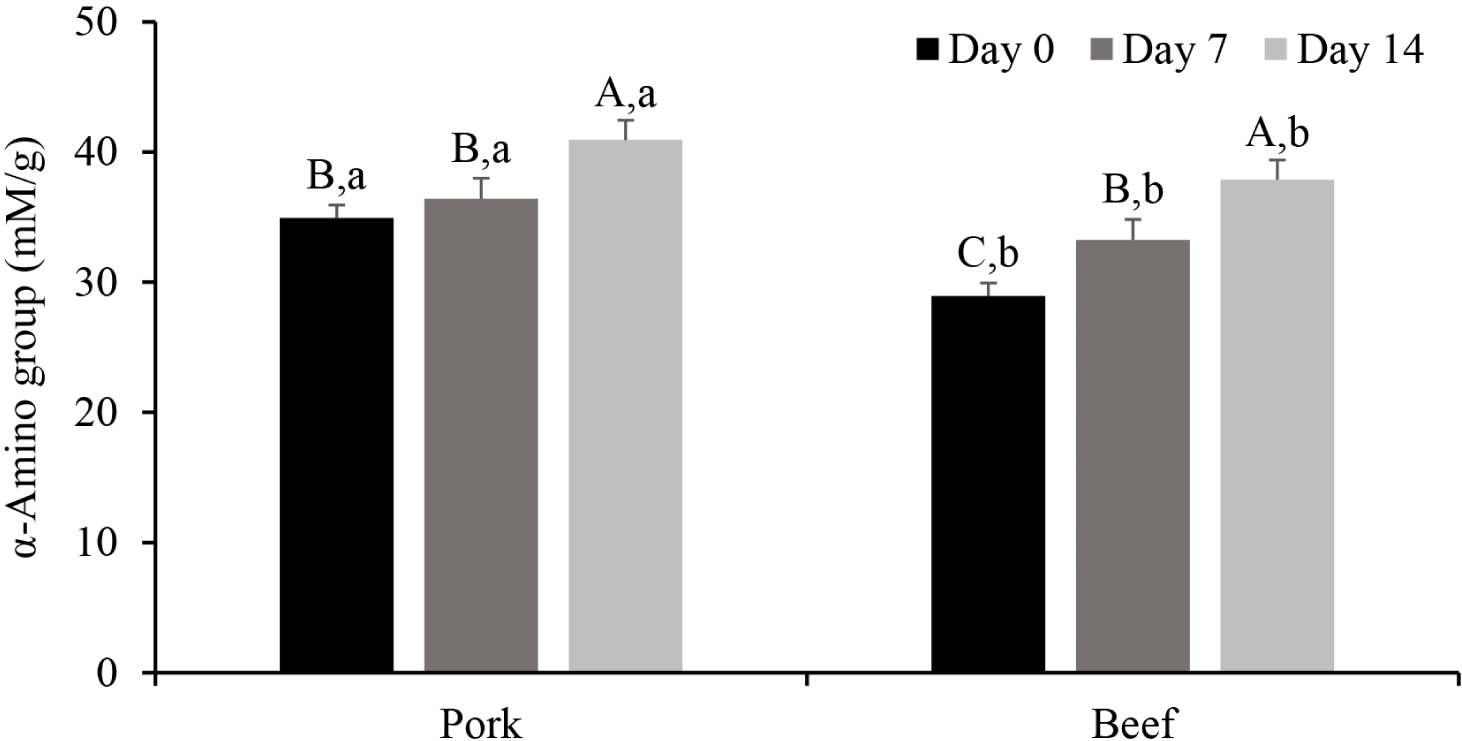
Despite the decreased calpain activity on Day 7, the protein degradation in beef increased on Day 7 and 14, where cathepsin B may have played a significant role in this outcome. The electrophoretogram of myofibrillar protein in beef (Fig. 4) revealed a 30 kDa band on Day 14, identified as the degradation product of troponin T, which is a primary substrate of cathepsin B (Bhat et al., 2018). This band, considered an indicator of meat protein degradation (Bhat et al., 2018), suggests that the continuous increase in small peptides and the appearance of troponin T degradation products in beef were primarily attributed to cathepsin B activity.
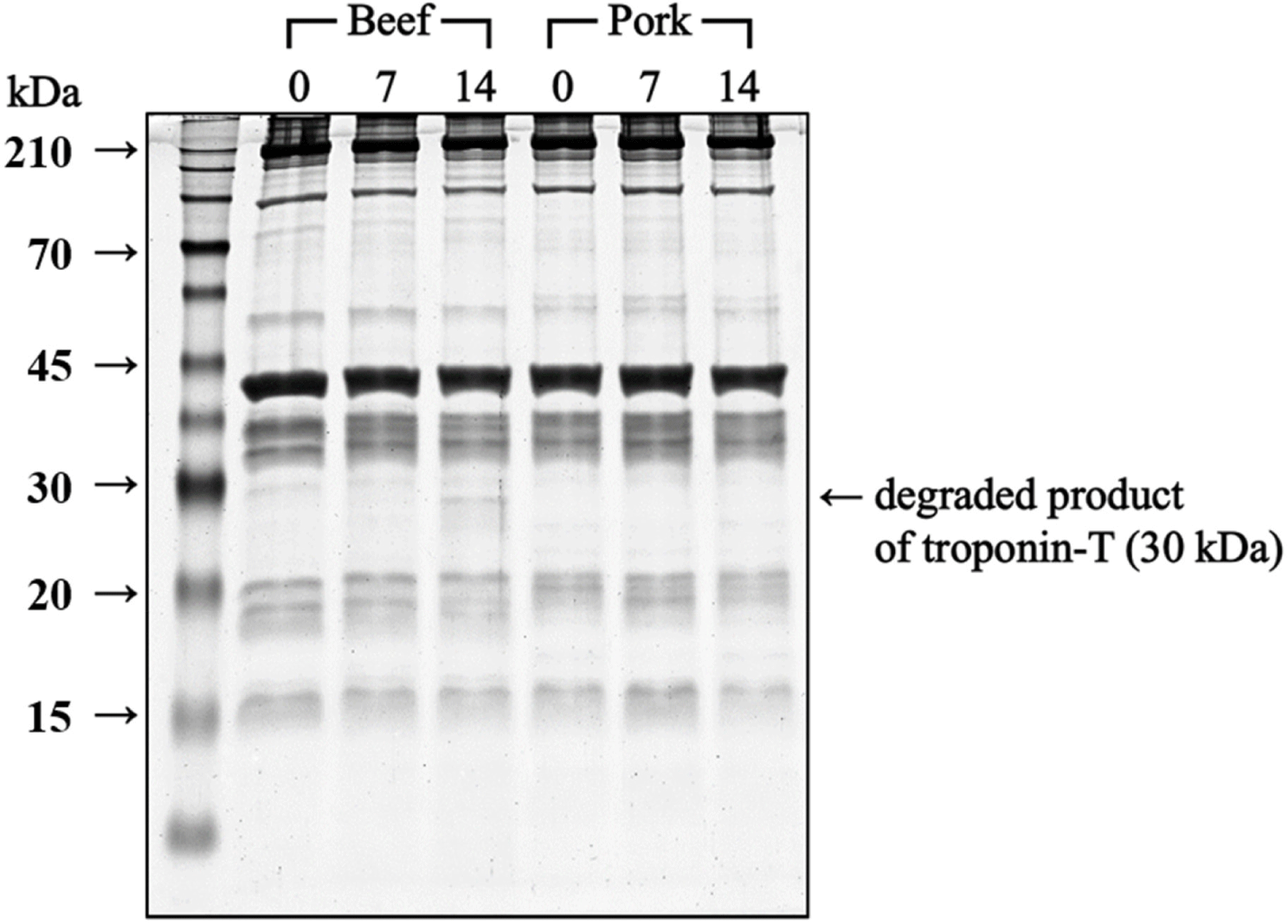
Overall, calpain in pork and cathepsin B in beef seemed to play significant roles in postmortem protein degradation. The data also confirmed gradual protein degradation during aging in beef, unlike pork with a higher α-amino group content on Day 0. Because the rate of protein degradation differs for the two species, the textural changes in beef and pork during aging differ.
The shear force in meat is commonly used as an indicator to evaluate tenderness; a lower shear force means that less force is required to cut the muscle fibers vertically, indicating higher tenderness (Novaković and Tomašević, 2017). Evaluation of the shear force after cooking beef and pork (Fig. 5A) indicated that there was no significant difference in the shear force of pork throughout the storage period (p>0.05), whereas the shear force in beef decreased on both Day 7 and 14 compared to Day 0 (p<0.05). These results indicate that an improvement in the tenderness due to aging was observed only in beef.
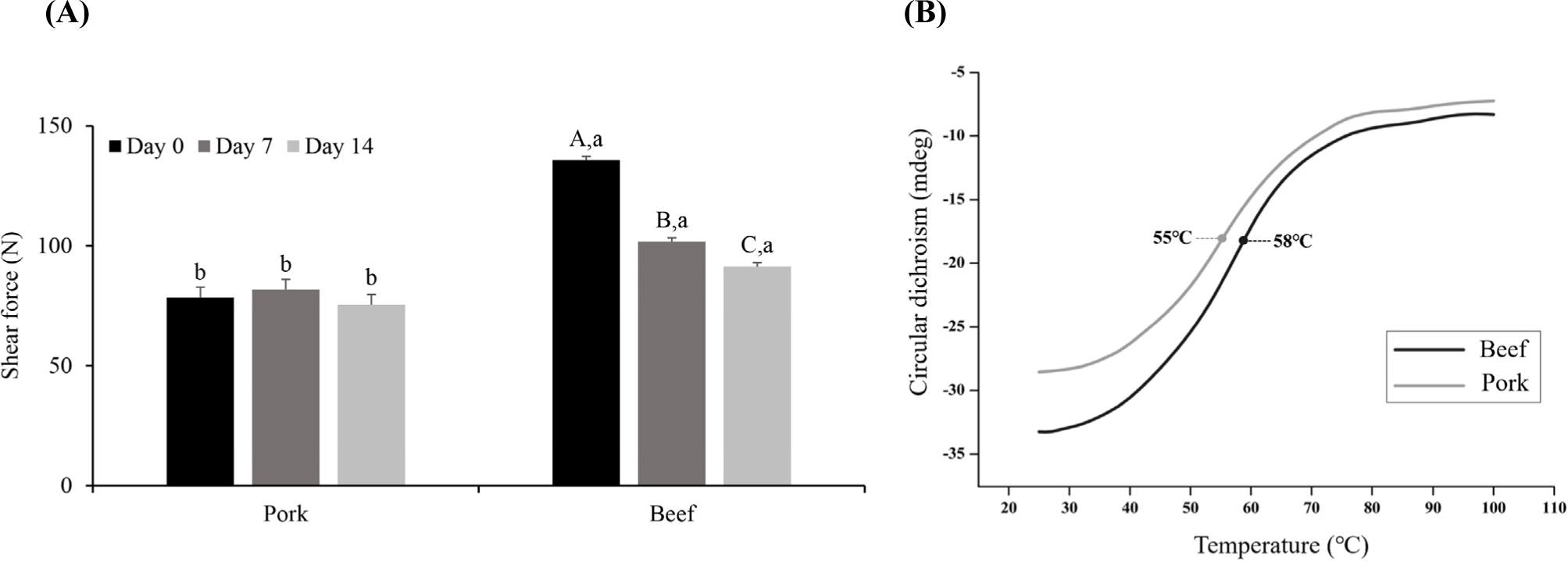
The decrease in the meat shear force during aging is mainly caused by the loss of the structural integrity of the muscle fibers due to protein degradation by endogenous proteolytic enzymes (Bhat, 2018). Analysis of the α-amino group content of the 10% TCA-soluble fraction and the SDS-PAGE study confirmed that protein degradation gradually occurred throughout 14 days of aging in beef, and the degradation product of troponin T appeared. Therefore, the muscle structure in beef may have been weakened by protein degradation, leading to improved tenderness.
In pork, no improvement in the shear force was observed on Day 14, whereas the protein degradation increased. In a study by Hamill et al. (2012), type IIB muscle fibers were negatively correlated with tenderness. In this study, the samples were cooked until the core temperature reached 75°C before measuring the shear force. During meat cooking, the structure of protein molecules changes from the native state to a less-folded state owing to thermal denaturation (Bax et al., 2012). Gelation occurs because of aggregation of the myofibrillar proteins. The temperature at which heat-induced denaturation is initiated varies depending on the type of muscle fiber (Vaskoska et al., 2021; Young et al., 1992). Myosin is responsible for the gelation of meat proteins (Sun and Holley, 2011). When thermal denaturation occurs, myosin heads are aggregated by disulfide bonds, resulting in a structural change in which the helical myosin rods are changed into β-sheets and random coils (Sun and Holley, 2011). Therefore, herein, the CD signal of the myosin extract was acquired at 222 nm for quantifying the α-helix content to determine the protein denaturation temperature (Shimada et al., 2015). The data confirm that the temperature at which denaturation occurred in the secondary myosin structure in pork and beef differed (Fig. 5B). The temperature at which the myosin α-helix is attenuated in pork and beef was confirmed to be 55°C and 58°C, respectively, consistent with the literature value for the thermal denaturation temperature of myosin of 50°C-60°C (Liu et al., 2021). The myosin extract of beef semitendinosus muscle had a higher thermal denaturation temperature than that of pork semitendinosus muscle (p<0.05). Vaskoska et al. (2021) reported that thermal denaturation occurred more quickly in muscle primarily composed of white muscle fibers than in muscles primarily composed of red muscle fibers. They also reported that the extent of protein aggregation after cooking was greater in muscles mainly composed of white muscle fibers, indicating lower thermal stability than that of red muscle fibers. Young et al. (1992) also reported that the temperature at which myofibrillar protein gelation initiates is 10°C lower in white muscle fibers than in red ones. Therefore, despite the occurrence of protein degradation during aging, the lack of improvement in the tenderness of pork after cooking may be due to greater protein aggregation than in beef during heating.
Pork had a lower shear force than beef during the entire aging period (p<0.05). It is known that the three most influential factors affecting the shear force of meat are the collagen content, sarcomere length, and proteolytic enzyme activity (Bhat et al., 2018). According to Bhat (2018), a high proteolytic enzyme activity, low collagen content, and long sarcomere length are required for high tenderness. In previous studies comparing the collagen content of the semimembranosus muscle in pork and beef, the collagen content in pork was reported to be 4.13 and 5.30 mg/g, while the collagen content in beef was reported to be 6.85 mg/g (Moon, 2006; Semenova et al., 2023; Seong et al., 2009). Additionally, in a study comparing the length of sarcomeres, the reported sarcomere lengths of pork and beef were 2.50 μm and 1.89 μm, respectively (Moon, 2006). Therefore, the low shear force of pork is considered to be due to its low collagen content and long muscle fiber length.
Overall, although the meat proteins in both pork and beef were degraded during aging, no improvement in the tenderness was observed in pork, potentially because of the lower thermal stability of the proteins and greater protein aggregation compared with that in beef during heating. In addition, the shear force of both meats differed on Day 0, potentially due to the different characteristics of pork and beef muscles (collagen content and sarcomere length). Although the improvement in the tenderness of pork was not significant, protein degradation during aging can improve other sensory qualities such as the flavor and taste by releasing free amino acids (Lee et al., 2020), and it is also expected that bioactive peptides released during aging can exhibit several biological effects (Zou et al., 2020).
Conclusion
This study examined proteolytic changes and shear force in pork and beef semitendinosus muscles during aging. Electrical conductivity increased on Day 7 for pork and Day 14 for beef postmortem. Calpain activity rose over Day 14 in pork but declined by Day 7 in beef. Cathepsin B activity increased on Day 14 in both species. α-Amino group content in the 10% TCA-soluble fraction increased on Day 14 and gradually during aging in pork and beef, respectively. A distinctive 30 kDa protein band (troponin T degradation product) was only observed in beef. Although pork had a lower shear force than beef throughout aging, a decrease in the shear force was only observed in beef. Different proteolytic patterns or thermal denaturation temperatures of myosin depending on the muscle fiber types can impact the tenderness of aged meat.
In conclusion, muscle fiber composition and resulting physicochemical properties should be considered for optimal meat textural quality through aging. Future studies are further required to comprehensively compare the proteolytic behaviors of the muscles depending on their primary types of muscle fibers and species.