Introduction
The principle aim of the meat industry is to produce safe products while improving sensory, texture, and quality properties and ensuring oxidative stability (Dominguez-Hernandez et al., 2018). The effects of freezing, which increases shelf life by preventing oxidative degradation, on product quality has been extensively studied (Choi et al., 2017; Leygonie et al., 2012a; Soyer et al., 2010). Tenderness and juiciness, which comprise crucial properties for consumers, exhibit more positive results upon freezing/thawing rather than chilling meat (Lagerstedt et al., 2008). However, the question has been raised regarding whether freezing/thawing leads to reduced meat quality (Kim et al., 2018). Ice crystal formation in muscle fiber destroys structural and nutrient homeostasis by inducing increases in protein, carbohydrate, lipid, vitamin, and mineral concentrations (Leygonie et al., 2012a). The damaged muscle fibers do not reabsorb water during the thawing process, leading to an overall increase in discoloration and decreased water holding capacity (WHC); lipid and protein oxidation also occur (Ali et al., 2015; Leygonie et al., 2012b).
High temperature cooked meat from the frozen or thawed state did not influence cooking yield and tenderness (Smith et al., 1969), but enhanced yield and juiciness (Jakobsson and Tsson, 1973). Alternatively, sous-vide processing transfers heat from water to food to uniformly cook the product (Jeong et al., 2018a), reduces moisture loss and heat damage to protein and lipids, and improves texture compared to traditional cooking methods (Sanchez del Pulgar et al., 2012; Jeong et al., 2018b). This processing is also referred to as vacuum packaged cooked foods, which are cooked for a long time at 65°C to 80°C to preserve flavor, texture, and nutritional characteristics (Gonzalez-Fandos et al., 2005).
Although cooking meat directly in the frozen or frozen/thawed state is controversial owing to quality degradation, the sous-vide method may represent a crucial alternative for quality improvement. This study was intended to analyze the pork quality, stability, texture, and sensory characteristics of longissimus dorsi muscle based on storage state and sous-vide cooking temperature.
Materials and Methods
A total of five pork loins (longissimus dorsi muscle) from the same side of the porcine carcass (n=5) was collected at 24 h postmortem from a local slaughter house. After trimming, they were equally cut into six 25 mm thick and about 95 g pieces, individually vacuum-packaged at settings of vacuum 30 s, sealing 3 s, and soft air 2 s (Cryovac C5045, nylon/PE/nylon/PE/ nylon/LLDPE, Sealed Air Corporation, Duncan, SC, USA), and randomly assigned into six treatments according to storage state {chilled (CS), frozen (FS), or frozen/thawed (TS)} and cooking temperature (1 h at 65°C or 72°C). All groups were cooled in a refrigerator for 1 h after cooking (Fig. 1). During the cooking process, the core temperature of samples was recorded using a thermocouple (Testo108, Testo, Lenzkirch, Germany) every 5 min (Fig. 2).
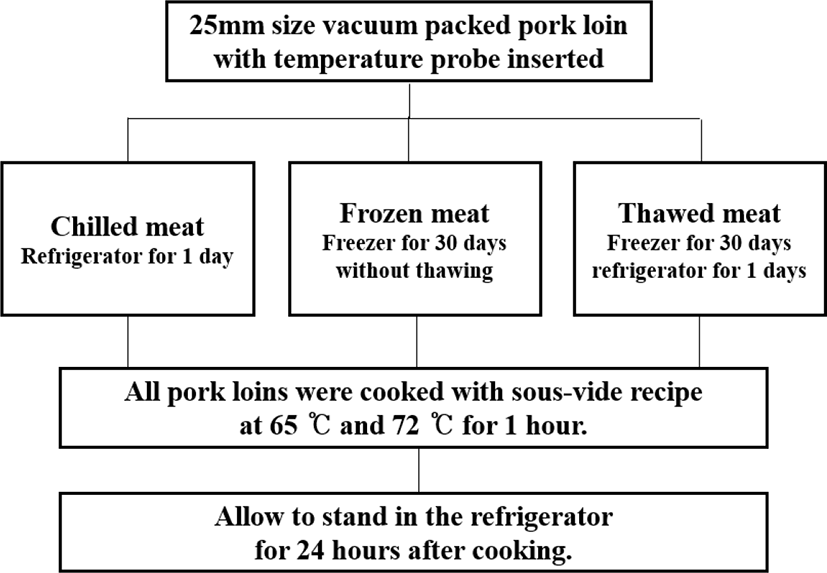
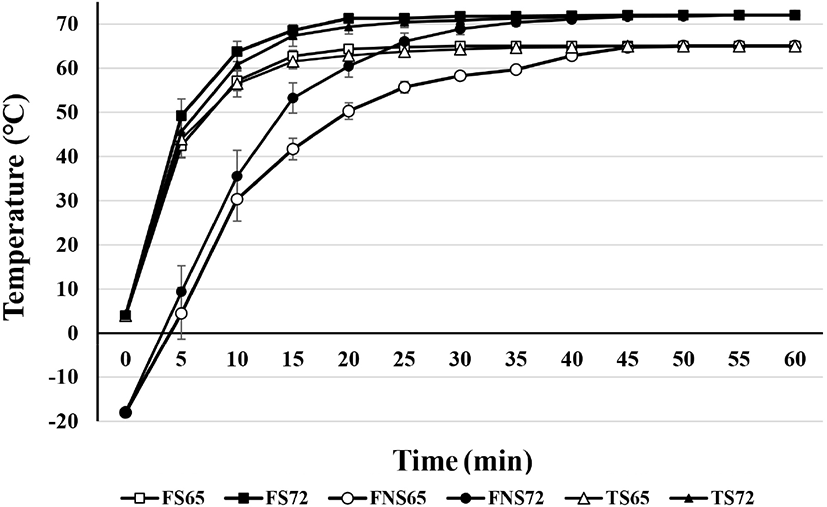
Proximate analysis was determined as moisture, crude fat, and crude protein contents (AOAC, 1995). Sample pH was estimated using a pH meter (F-71G, Horiba Co., Kyoto, Japan) after pulverizing a 2 g sample with 18 mL distilled water for 1 min in a homogenizer (AM-1, Nihonseiki kalsha, Tokyo, Japan).
For expressible moisture, 1.5 g was wrapped in gauze, placed in a conical tube, then centrifuged at 1,000×g, 15 min (VS-550, Vision, Daejeon, South Korea). The weight of expressible moisture was calculated according to the following equation:
Cooking loss was measured by weight difference between the pork loin before and after cooking and calculated according to the following equation:
The sample was assessed after sous-vide cooking and 1 h refrigerator cooling. The inside color of meat was estimated using a colorimeter (Chromameter Minolta Co., Japan) using by the Commission International de1’Eclairage (CIE) system. The colorimeter was calibrated employing a standard white plate (CIE L*=+97.43, CIE a*=−0.08, CIE b*=+1.83).
TBARS was measured as previously described (Buege and Aust, 1978) based on the malondialdehyde (MDA) amount. Sous-vide-treated sample (5 g) was added to 15 mL distilled water and 100 μL butylated hydroxytoluene (6%, v/w). After homogenizing for 1 min at 3,220×g, 1 mL sample was reacted with 2 mL of 20 mM TBA/15% trichloroacetic acid reagent (TCA, v/w), vortexed, heated in an 80°C water bath for 15 min, cooled for 30 min in ice water, centrifuged (2,000×g, 10 min, 25°C), and filtered through Whatman paper (No. 1). The absorbance was analyzed using a spectrophotometer (Optizen 2120UV, Mecays, Seoul, Korea) at 532 nm (A532). TBARS number was calculated as mg MDA/kg meat using a mole extinction coefficient of 1.56×105 M−1cm−1. The MDA concentration was calculated according to the TBARS number per the following equation:
Protein oxidation measurements were based on carbonyl and sulfhydryl content (Vossen and De Smet, 2015). For carbonyl content, the sample (3 g) was homogenized with 20 mM phosphate buffer (30 mL) containing 0.6 M NaCl. To each sample, 10% TCA regent (v/w) was added, held for 30 min in an ice bath, centrifuged (2,000×g, 30 min), the supernatant discarded, 10% TCA reagent again added, and the process repeated. DNPH reagent was then added to the sample, the mixture shaken for 1 h in a dark room, supplemented with 20% TCA (0.5 mL, v/w), the cooled sample centrifuged (2,000×g, 20 min), and the supernatant removed. The remaining pellet was washed three times with added 1 mL ethanol/ethyl acetate solution (1:1, v/v), centrifuged (2,000×g, 20 min), the supernatant removed, the solvent evaporated for 15 min, then 1 mL guanidine-HCl (6 M) in 20 mM phosphate buffer was added, mixed for 30 min, and centrifuged (9,500×g, 10 min). The absorbance was measured at 280 nm for measuring protein concentration using the bovine serum albumin (BSA) standard curve and 370 nm for measuring protein hydrazones using a nanomole extinction coefficient of 0.021 nM−1cm−1; the carbonyl content was expressed as nmol carbonyl/mg protein.
For sulfhydryl content, 2 g sample was added to 5% SDS buffer (50 mL), homogenized, placed in a tube, heated in a water bath at 80°C for 1 h, cooled for 30 min, and filtered using Whatman paper (No. 1). Then, 0.1 M TRIS buffer (2 mL, pH 8.0) and 10 mM DTNB (0.5 mL) were added to 0.5 mL filtered sample, reacted in a dark room for 30 min, and the absorbance of sulfhydryl content was analyzed at 412 nm. Sulfhydryl content was calculated as a nanomole extinction coefficient of 0.0114 nM−1cm−1 and protein content was measured based on the BSA standard curve using the Biuret method; the sulfhydryl content was expressed as nmol sulfhydryl/mg protein.
The total protein solubility was analyzed according to the method of Bowker and Zhuang (2016). Potassium phosphate buffer (0.025 M, pH 7.2) and 0.55 M potassium iodide in 0.05 M potassium phosphate buffer (pH 7.2) were respectively used to measure sarcoplasmic and total protein solubility. Cooked sous-vide samples (1 g) were added to 10 volumes of each reagent, homogenized at 3,220×g for 1 min, and stored in a refrigerator for 20 h. After centrifugation (2,600×g, 30 min, 4°C), the protein in the supernatant was quantified using the Biuret test. The following equation was used without measuring the myofibrillar protein solubility (myofibrillar protein=total protein–sarcoplasmic protein). Protein solubility was given as mg soluble protein per g meat.
Shear force and texture profile were measured using a TX-XT2 instrument (Stable Micro Systems Ltd, Surrey, England). For the shear force, the cooked pork loin sous-vide sample was cored to a cylindrical 12 mm diameter. Operating parameters were 5 kg load cell and 200 mm/min cross-head speed. Shear force value was calculated by taking the average of the maximum force required to shear the cored sample. Texture analysis conditions were pre-test speed 2.0 mm/s, post-test speed 5.0 mm/s. maximum load 2 kg, head speed 2.0 mm/s, distance 6.0 mm, trigger force 5 g. The central portion of sous-vide cooked longissimus dorsi muscle was cut to a 10×10×15 mm sample, which was placed under the probe moving down at a constant speed; results were automatically recorded by the software. Texture parameters for hardness (kg), springiness (mm), gumminess (kg), and cohesiveness and chewiness (kg) were assessed using the method of Bourne (1978).
Stored samples (about 95 g) were cooked in sous-vide processing for 1 h at 65 and 72°C, then cooled at room temperature for 30 min. The samples were cut to a 10×15×15 mm block and placed on a white plate. The sensory analysis was performed by ten untrained consumer panelists (6 women and 4 men aged 25 to 34 years with an average age of 29 years). The sensory analysis conducted twice, once in the morning and once in the afternoon with the same panelists. At each session, each sample was provided with a random three-digit number. Each untrained consumer panelist rated six samples using a hedonic seven-point scale of lightness, redness, flavor, odor, tenderness, juiciness, and overall rating: 1 (strongly non-like) to 7 (strongly like). Untrained consumer panelists for this study were selected by the Konkuk University Meat Science Laboratory.
The factorial design was conducted for each sample status (chilled, frozen, and frozen/thawed) and two cooking temperatures (65°C and 72°C), where each carcass served as a block. All methods were analyzed by two-way ANOVA procedure of IBM SPSS statistics 24.0. Difference significant between storage state (chilled, frozen, and frozen/ thawed), temperature (65°C and 72°C) and storage×temperature (S×T) interaction effects were used to identify (Tukey’s HSD test, p<0.05).
Results and Discussion
Table 1 reveals the effect of storage state and sous-vide cooking temperature on proximate composition and pH of pork. The crude protein and fat were not influenced by storage state, cooking temperature, or S×T interaction. The FS showed higher moisture content than those of TS and CS (p<0.001). The moisture contents influenced by the holding time of sous-vide cooking procedures. Obuz et al. (2003) reported that short holding time resulted less cooking loss. When cooking loss is reduced, water may be captured more in the protein structure than the higher cooking losses, resulting in higher water content (Aaslyng et al., 2003). The moisture of the 65°C groups (66.07%) was significantly higher than that of the 72°C groups (63.02%). Previous study also reported that low sous-vide cooking temperature resulted low cooking loss and high moisture content (Sanchez del Pulgar et al., 2012).
a,b Different superscript letters within the same common category indicate significant differences for the storage state (p<0.05).
Meat storage groups: CS, chilled state on sous-vide; FS, frozen state without thawing on sous-vide; TS, frozen/thawed state on sous-vide.
The pH range was 5.91–5.98, which did not significantly differ by storage methods or sous-vide cooking temperatures. Muela et al. (2010) stated that the pH of fresh, frozen, and thawing samples did not significantly differ. When cooked at 61°C for 45 min, the pH was 6.02 (Jeong et al., 2018b), similar to the ranges of the present study. Overall, our findings indicated that the range of pH did not significantly differ between conditions, indicating that pH had no effect on frozen state and cooking temperature.
Fig. 3 reveals the effects of storage method and sous-vide cooking temperature on WHC (cooking loss and expressible moisture). Cooking loss is related to the juiciness of the meat product (Kerr et al., 2005), and FS showed lower cooking loss than that of TS and CS (p<0.001). Sous-vide treatment at 65°C showed lower cooking loss than that of 72°C (p<0.001). The changes of the water in muscle tissue is due to the heat-induced proteins denaturation and structural contraction (Ishiwatari et al., 2013). The low core temperature indicates the lowest cooking loss as a result of deteriorates the strength of the connective and less changes in myofibrillar protein (Becker et al., 2016). Furthermore, Aaslyng et al. (2003) reported that not only the cooking temperature but also the time that reached at cooking temperature and holding time affect the protein denaturation. The frozen state of sous-vide cooking had a short holding time because it reaches at the cooking temperature later than the chilled and frozen/thawing state of sous-vide cooking (Fig. 2). This results is similar to the previous study reported that short holding time showed low cooking loss (Obuz et al., 2003). In this study, the FS showed lower cooking loss than CS and TS and also the lowest cooking loss was observed in FS65. The low cooking sous-vide treatment in the frozen state can be expected to have higher eating quality with less cooking loss and high juiciness than that of the sous-vide treatment in chilled and frozen/thawed state.
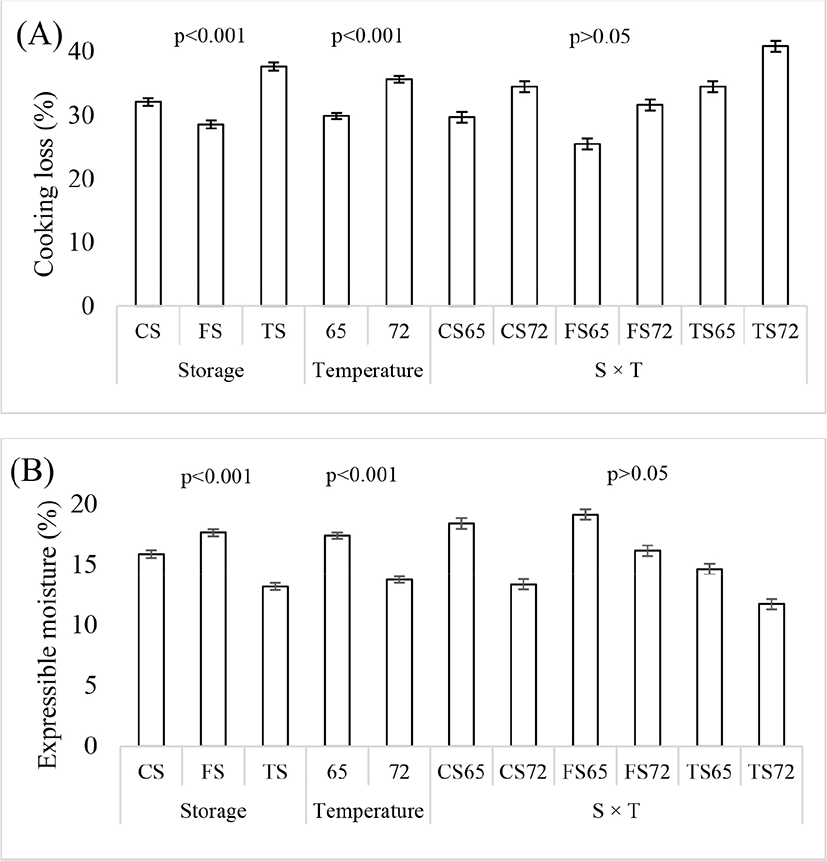
Expressible moisture is the measure of the juice preserved in the cooked product (Kerr et al., 2005). The FS expressible moisture was higher than that of TS and CS (p<0.001). Fernandez et al. (2007) reported the lower water release during centrifugation of expressible moisture resulting from water loss through the thawing process. In addition, Kerr et al. (2005) reported that expressible moisture may contain less juice that can be expressed after cooking due to loss of juice during cooking. Theses indicated that expressible moisture are inversely related to thawing and cooking loss. Therefore, frozen treatment and low cooking temperature treatment resulted in increase of WHC (low cooking loss and high expressible moisture).
Results of color measurement are revealed in Table 1. There was a significant effect on CIE L*, a*, and b* of S×T interaction, but not on that of storage groups (CS, FS, and TS). Dominguez-Hernandez et al. (2018) reported that the color remained constant when the core reached the final temperature through low temperature cooking. Therefore, sous-vide cooking was less affected in terms of color change and the cooking temperature groups (65°C and 72°C) were influenced by CIE a* and b* (p<0.001). As the cooking temperature increases, myoglobin is degraded and CIE a* is reduced (Roldan et al., 2013). CIE b* is increased owing to the thermal denaturation and the formation of metmyoglobin; accordingly, the loss of CIE a* and the increase of CIE b* were found by other authors as the sous-vide cooking temperature increased (Roldan et al., 2013; Sanchez del Pulgar et al., 2012).
As shown Table 2, TBARS, a measure of the amount of MDA as a secondary lipid oxide (Buege and Aust, 1978), was significantly (p<0.001) elevated CS<FS<TS (0.13, 0.32, and 0.40 MDA mg/kg, respectively). Higher oxidation level was influenced to accumulate unstable MDA because of cell membrane damage owing to ice crystals, especially heme iron release (Coombs et al., 2018; Leygonie et al., 2012a). Leygonie et al. (2012a) reported that when stored at −20°C, water did not freeze completely, and that freezing led to primary peroxidation whereas thawing promoted a radical secondary lipid oxidation. In the present study, TBARS was influenced by cooking temperature (p<0.05) but not by S×T interaction (p>0.05). Heat treatment increases the lipid oxidation of meat, and the oxidation rate may increase when the cooking temperature increases (Roldan et al., 2013). Oxidation is considered to be the cause of deterioration of taste, flavor and meat quality (Scollan et al., 2014). However, all treatment groups (CS65, CS72, FS65, FS72, TS65, and TS72) were within the acceptable edible range of 1.2 mg MDA/kg meat (Kim et al., 2015). These results indicate that changes in storage method and sous-vide cooking temperature are not negative effect on the lipid oxidation.
a–c Different superscript letters within the same common category indicate significant differences for the storage state (p<0.05).
Meat storage groups: CS, chilled state on sous-vide; FS, frozen state without thawing on sous-vide; TS, frozen/thawed state on sous-vide.
Carbonyl and sulfhydryl content were not influenced by storage and cooking temperature (p>0.05, Table 2). The interaction of S×T had no difference in carbonyl and sulfhydryl content (p>0.05). The first event observed during the protein oxidation process is that the sulfhydryl group is converted to disulfide and other oxidized species, resulting in reduced sulfhydryl content, and when MDA forms a protein complex, its carbonyl content increases (Xia et al., 2009). Loss of sulfhydryl content and formation of carbonyl content were not promoted; similarly, previous results showed that sulfhydryl and carbonyl content did not significantly differ after freezing for 3 months (Soyer et al., 2010). In addition, protein oxidation induced protein cross-linking, especially myofibrillar protein aggregates (Laakkonen et al., 1970; Xia et al., 2009). Table 2 shows that the myofibrillar protein did not aggregate and or show changes in physical structure, which would not be influenced by protein oxidation (carbonyl and sulfhydryl content). In conclusion, protein oxidation is stable in 1 month-frozen meat upon processing by sous-vide cooking.
Table 2 reveals the effect of storage state and sous-vide cooking temperature on total, sarcoplasmic, and myofibrillar protein solubility. Total and myofibrillar protein solubility did not differ by S×T interaction and storage (p>0.05). Protein solubility is widely used as an indicator of protein structural changes (e.g., protein denaturation), as the solubility is decreased owing to the generation of non-extractable insoluble protein aggregates (Laakkonen et al., 1970; Marcos et al., 2010). Sarcoplasmic protein solubility is a great indicator of quality and a significant part of meat processing (Marcos et al., 2010). Dai et al. (2013) reported that the solubility of sarcoplasmic protein is influenced by WHC, shear force, and gel formation. The decreases in sous-vide cooking temperature significantly increase the solubility of sarcoplasmic and total protein (p<0.001). These results showed the same results as the previous studies (Li et al., 2013; Murphy and Marks, 2000). High protein solubility can be result the less insoluble protein aggregates, increased WHC and tenderness compared to lower protein solubility (Dai et al., 2013; Marcos et al., 2010).
Table 3 shows the effect of storage state and sous-vide cooking temperature on shear force. Tenderization is determined to result from structural destruction owing to the damage caused by ice crystal formation (Lagerstedit et al., 2008). Shear force was significantly lower (p<0.001) in FS and TS groups (3.90 and 3.45 kg, respectively) than the CS group (4.65 kg), whereas S×T interaction had no effect (Table 3). Destefanis et al. (2008) reported that the perception of tenderness is defined as the range of shear forces (from 3.36 to 4.36 kg tender, from 4.37 to 5.2 kg tough). In previous studies, the frozen and frozen/thawed samples showed lower shear force tendency than the refrigerated samples (Leygonie et al., 2012a; Shanks et al., 2002). Sous-vide treatment at 65°C (3.60 kg) was lower a shear force than that of 72°C (4.40 kg). Garcia-Segovia et al. (2007) reported that the sous-vide cooking from 60°C to 80°C increased shear force value, but Vaudagna et al. (2002) reported that at the 60°C to 65°C the meat was maintain as tenderness. This result is based on myosin (55°C–60°C) and actin (about 80°C) denaturation and collagen contraction (56°C–65°C), as the temperature decreases, the shear force decreases due to less collagen denaturation and myofibrillar structure (Roldan et al., 2013; Garcia-Segovia et al., 2007). In particularly, low shear forces were showed in FS65 and TS65. As the previous studies reported, the freezing process and low cooking temperature tended to decrease the shear force (Lagerstedit et al., 2008; Shanks et al., 2002; Garcia-Segovia et al., 2007).
a,b Different superscript letters within the same common category indicate significant differences for the storage state (p<0.05).
Meat storage groups: CS, chilled state on sous-vide; FS, frozen state without thawing on sous-vide; TS, frozen/thawed state on sous-vide.
Table 3 reveals TPA values by storage method and cooking temperature. The high moisture content shrinkage the muscles in the meat and influence the TPA parameter (springiness, gumminess, cohesiveness, hardness, chewiness) (Du and Sun, 2005). In this study, cohesiveness, hardness and chewiness did not show significant difference for storage, cooking temperature and S×T. Gumminess of FS was lower than those of CS and TS, and springiness of 65°C was lower than that of 72°C. In Table 1, FS showed higher moisture content than TS and CS, which is consistent to the previous study that reported high water content resulted low gumminess (Zhang and Barbut, 2005). Moreover, previous study indicated that heating above 65°C increase the modulus of elasticity (Tornberg, 2005).
Table 4 shows the effect of storage state and cooking temperature on sensory analysis. Tenderness, juiciness, and flavor were the main factors of consumer's choice of meat (Dominguez-Hernandez et al., 2018). Tenderness scores of FS and TS groups (5.92 and 5.67, respectively) were higher than that of the CS group (4.53, p=0.001); moreover, tenderness of 65°C was higher than that of 72°C. Shear force generally has high correlation with sensory scores on meat toughness (Tornberg, 2005), consistent with sensory results and shear force measurements. The FS and TS showed higher juiciness than the CS group (p=0.001), with juiciness of the 65°C group being higher than that of the 72°C group (p<0.001). Aaslyng et al. (2003) reported that low cooking loss result the high juiciness. Accordingly, FS65 showed the lowest cooking loss (Fig. 3) and showed the highest juiciness. The FS65 showed the highest score in flavor, tenderness and overall as well as juiciness. This results suggest that frozen state and low sous-vide cooking have a positive effect on sensory quality.
a,b Different superscript letters within the same common category indicate significant differences for the storage state (p<0.05).
Meat storage groups: CS, chilled state on sous-vide; FS, frozen state without thawing on sous-vide; TS, frozen/thawed state on sous-vide.
Conclusion
This study investigated the meat quality effect on the storage state and the cooking temperature. Frozen state on sous-vide treatment increased the moisture content and improve the sensory quality (tenderness and juiciness) and decreased cooking loss. Moreover, frozen and frozen/thawed on sous-vide treatment showed lower shear force than chilled on sous-vide treatment. This study suggests that frozen state on sous-vide cooking may minimize the quality degrenation. In addition, sous-vide cooking temperature at 65°C showed higher moisture content, sarcoplasmic protein solubility, tenderness and juiciness and lower shear force and cooking loss than that of 72°C. Therefore, the combination of frozen state and 65°C sous-vide cooking temperature may produce the high quality meat products with the advantages of high moisture content, WHC, tenderness and juiciness and low share force. However, further study is necessary to indicate the effect of storage state and sous-vide cooking temperature on oxidation stability of the meat products.