Introduction
Dietary fat is a major nutritional and biochemical component of the human body necessary for maintaining physiological, biochemical, and molecular mechanisms and the composition of cell components and for providing a source of energy. As a dietary component, fat plays a major role in taste, flavor, and aroma in most foods. In contrast, excessive fat consumption has been associated with the occurrence of chronic human diseases, such as obesity, cardiovascular disease, diabetes, and cancer (Hariri and Thibault, 2010). Thus, efforts to reduce total fat consumption have continued for decades. However, according to recent reports from the National Health and Nutrition Examination Survey, the incidence of chronic diseases has remained high, despite reductions in fat intake (Austin et al., 2011). Therefore, recent studies have recommended that people should consider improving the quality of fat consumed, instead of reducing total fat intake, because the balance of health benefits to risks is determined by the amount of saturated fatty acids (SFA) and unsaturated fatty acids (USFA) consumed (Moro and Capel, 2019). Current recommendations limit SFA consumption to less than 10% of total calories due to the risk of cardiovascular disease (USDA and HHS, 2015). In contrast, USFA consumption showed positive health effects through decreased low-density lipoprotein cholesterol levels and reduced coronary heart disease (Li and Sun, 2019). Thus, the consumption of unsaturated fats (e.g., vegetable and fish oil) has been recommended, instead of saturated fats (e.g., lard and tallow), to reduce adverse health effects (Moghtadaei et al., 2018).
Duck meat is a type of poultry meat with a unique flavor. It also contains high levels of essential amino acids and polyunsaturated fatty acids (Jo et al., 2018). Particularly, a higher amount of long-chain fatty acids, such as oleic acid (C18:1) and linoleic acid (C18:2) in duck skin and tissue, have been reported (Chen et al., 2017; Heo et al., 2013). In fact, these fatty acids have been known to prevent cardiovascular disease, including arteriosclerosis and cholesterol and triglyceride accumulation in blood vessels. It was estimated that the worldwide duck meat consumption is approximately 4.53 million metric tons (MMT) per annum, while poultry meat accounts for 121 MMT per annum (FAOSTAT, 2018; Khan et al., 2019). The increased production and consumption of duck meat has resulted in large amounts of by-products, such as duck skin. Duck skin fat has the potential for use as a raw material in the production of healthy animal-derived fats (Huda et al., 2013).
Pressurized hot water extraction (PHWE), a process which combines pressure and high water temperature, is a green extraction method for foods and herbal plants (Chemat et al., 2012). The advantage of PHWE is that the degradation of minor components is minimized because of the short extraction time (dos Santos Freitas et al., 2008). More importantly, PHWE utilizes hot water, whereas traditional extraction methods, such as pressurized liquid extraction requires non-environmentally friendly organic solvents (Kronholm et al., 2007). Studies on the quality properties of vegetables and fruit oil prepared by PHWE have been published (Mustafa and Turner, 2011). PHWE-employed fat extraction from animal skin has not been frequently used but its advantages to protect minor components in the fats and protect the environment warrant further investigation.
Therefore, in the current study, the oxidative stability and quality characteristics of duck skin fat were evaluated in comparison with those of other animal fats (chicken, swine, and bovine skin fats). An identical fat extraction method (i.e., PHWE) was used for all animal skins. This study was also performed to provide information on the potential use of duck skin fats as a healthy edible animal fat in the food industry and for cooking.
Material and Methods
Potassium iodide, p-anisidine, potassium hydroxide, heptadecanoic acid methyl ester, starch, thiobarbituric acid, and phenolphthalein were purchased from Sigma Aldrich Chemical Co. (St. Louis, MO, USA). Chloroform, isooctane, glacial acetic acid, methanol, sodium thiosulfate (0.1 N and 0.01 N), ethanol, and ether were purchased from Daejung Co. (Siheung, Korea). Benzene and iodine monochloride were purchased from Samchun (Seoul, Korea). All chemicals were analytical grade and used without further purification.
Animal skins (duck, chicken, swine, and bovine skin) were obtained Farm Duck Co. Ltd., Jeongeup-si, Korea). The PHWE method has previously been reported for animal fat extraction (Plaza and Turner, 2015). In detail, animal skins (2 kg) were washed several times and the visible fat and connective tissue were removed from the skin. Fat extraction was performed using a pressure extractor (Yu-il ENT Co. Ltd, Seoul, Korea) at 115°C, 1.4 kgf/cm2 for 3 h and dehydration for 30 min. The samples were filtered using a 40-mesh bucket filter, then the filtrate was centrifuged using a decanter (GEA Westfalia Separator, Oelde, Germany) at 7,500 rpm for one hour. The fat phase was collected from the supernatant and re-centrifuged at 15,000 rpm for one hour. The extract was packed in a plastic container and stored at –80°C until use.
A modified Schaal oven test (accelerated storage test) was used to measure the oxidative stability of fat at 60°C (Warner et al., 1989). Briefly, the extracted fat samples (1 kg) were poured into glass beakers and placed in a drying oven (SW-90D, Sangwoo Scientific Co., Korea) which was set to 60±2°C for 90 days. Experimental analyses were performed within a 10-day interval.
The extract yield was determined by calculating the difference in the weights of the samples before and after processing:
The fatty acid composition was determined using the method reported previously by Muguerza et al. (2001). Briefly, the fatty acid composition was determined using gas chromatography (5890, Agilent Technologies, Santa Clara, USA) with a capillary column SP-2560 (100 m×0.25 mm×0.2 μm). Chromatographic conditions were as follows: the temperature of both the injector and detector was 225°C, the oven temperature was programmed to increase from 100°C to 240°C at a rate of 1°C/min. Hydrogen was the carrier gas at a flow rate of 1 mL/min. The quantification of individual fatty acids was determined by using heptadecanoic acid methyl ester as an internal standard.
The iodine value was measured as described previously (Kyriakidis and Katsiloulis, 2000). The samples (0.2–0.4 g) were dissolved in 20 mL of chloroform and 25 mL of iodine monochloride solution in a flask. The mixture was kept for 30 min in the dark at room temperature, then 20 mL of 1 N KI solution and 100 mL of distilled water was added. Then, the mixture was titrated against 0.1 N sodium thiosulphate solution with 1% starch solution as an indicator. IV was calculated with the following equation:
Where a was the volume (mL) of 0.1 N sodium thiosulfate consumed in the titration, b was the normality of the 0.1 N sodium thiosulfate, and W was the weight of the sample (g).
Free fatty acid content was determined by a standard titrimetry method (Ghadge and Raheman, 2005). The samples (5 g) were mixed with 100 mL of ethanol/ether solution (1:2, v/v) and the mixture was titrated against 0.1 N potassium hydroxide with 1% phenolphthalein as an indicator. Acid value (AV) was calculated with the following equation:
Where a was the volume (mL) of potassium hydroxide consumed in the titration, b was the normality of the potassium hydroxide and W was the weight of the sample (g).
The PV of the samples was determined by the AOAC (2007) method. The samples were dissolved with 10 mL of chloroform and 15 mL of glacial acetic acid in a flask. Then, 1 mL of saturated solution of KI was added to the flask and kept for 10 min in the dark. Samples added with 30 mL of distilled water were thoroughly mixed and the mixture was titrated against 0.01 N sodium thiosulphate solution with 1% starch solution as an indicator. The PV was calculated as follows:
Where a was the volume (mL) of sodium thiosulfate consumed in the titration, b was the normality of the sodium thiosulfate, and W was the weight of the sample (g).
Determination of the p-AVs was carried out as previously described (Hashempour-Baltork et al., 2017). Samples (1 g) were dissolved in isooctane in a 25 mL volumetric flask and mixed with 1 mL p-anisidine solution (0.25% w/v in 99.5% glacial acetic acid). The mixture was reacted for 10 min in the dark. The absorbance was measured at 350 nm using a UV/VIS spectrophotometer (Optizen 2120 UV Plus, Mecasys Co., Ltd., Daejeon, Korea) and the p-AV was calculated as follows:
Where, As was the absorbance of the sample with p-anisidine solution, Ab was the absorbance of the sample without p-anisidine solution, and W was the weight of the sample (g).
The total oxidation state (TOTOX value) of the oils and fats was calculated according to the formula:
The thiobarbituric acid reactive substances (TBARS) value was obtained following a procedure described previously (Papastergiadis et al., 2012). A 1 g sample was weighed into a 50 mL conical tube and mixed with 5 mL distilled water for two minutes. The mixture was centrifuged at 5,000 g for five minutes. The aqueous layer was collected, and the procedure was repeated twice. The collected sample (2.5 mL) and 2.5 mL of TBA reagent (46 mM in 99.5% glacial acetic acid) were mixed in a test tube and heated in a water bath at 95°C for 35 minutes. After cooling, the absorbance was measured at 532 nm using a UV/VIS spectrophotometer. The results were expressed as mg MDA/kg.
The conjugated dienes (CDs) were determined using a UV/VIS at 233 nm. Before analysis, 100 mg of sample was diluted with isooctane in a 25 mL of volumetric flask. The CD expressed as a percentage of conjugated dienoic acid was calculated as follows:
Where Ab233 was the absorbance observed at 233 nm, b was the cell length (cm), c was the concentration of the sample (g/L), and K0 was the absorptivity of the acid (value of 0.03).
The viscosity of the samples was analyzed using a DV-E viscometer (Brookfield, Toronto, Canada) during the storage period. Thirty mL of sample was added to a conical tube and the analysis was conducted at a constant rotational speed of 50 rpm using the No. 62 spindle. The measurement was repeated five times for 30 s and the results were expressed as centipoise (cP) units.
Statistical analysis was performed with two-way analysis of variance (ANOVA). The ANOVA was performed on all the variables using the General Linear Model (GLM) procedure of SPSS Ver. 24.0 (SPSS INC., USA). Duncan’s multiple range test (p<0.05) was used to determine significant differences between the sample groups.
Results and Discussion
The extraction yields of animal skin-derived fats using PHWE are shown in Table 1. The highest (p<0.05) fat extraction (60.73%) was obtained from bovine skin, followed by duck skin (34.05%), swine skin (23.20%), and chicken skin (14.52%). Bovine skin products contained the highest amount of fats, whereas chicken skin contained the lowest amount of fats. The IV represents the degree of unsaturated fats and oils and also indicates the relative content of USFA (Naz et al., 2005). The IV determines the quality and grade of oil and is used as an authentication test in the oil industry (Yan et al., 2018). The IV of animal skin fat in the present study is shown in Table 1. The IV of the samples ranged from 55.71 to 79.57 g I2/100 g. The IV of duck and chicken fats was 77.57 and 77.32 g I2/100 g, respectively. Fats containing high IVs are chemically unstable because the double bonds in USFA are relatively reactive and vulnerable to oxidation (Encinar et al., 2019). Duck fat has been characterized by a high ratio of USFA. The major fatty acids identified were palmitic acid (C16:0), stearic acid (18:0), oleic acid (C18:1), linoleic acid (C18:2), and arachidonic acid (C20:4) (Cobos et al., 2000). The higher IV duck skin fat observed in this study reflected its higher USFA contents.
The fatty acid composition of fat is a critical indicator of nutritional value. The fatty acid composition is associated with oxidative stability and thus, high levels of polyunsaturated fatty acids can contribute to the rapid deterioration of fats (Symoniuk et al., 2019). The fatty acid composition of the animal skin fats is summarized in Table 2. The fatty acid compositions were significantly different based on the origin of the animal skin fat (p<0.05). For most skin fats, oleic acid (C18:1) was the major USFA, followed by palmitic acid (C16:0), linoleic acid (C18:2), and stearic acid (C18:0). Only bovine skin fat showed more stearic acid (C18:0) than linoleic acid (C18:2). The fatty acid composition measured in the skin of animals were similar to previously reported data (da Silva et al., 2019; Kang et al., 2014; Marion and Woodroof, 1963; Park et al., 2014). In the current study, duck skin fat displayed a higher content of oleic acid (p<0.05) than other skin fats. Oleic acid plays an important role in human nutrition (Rekas et al., 2015). Particularly, oleic acid is an USFA that provides biologically beneficial effects in the human body. A previous report demonstrated that the consumption of canola oils enriched with high oleic acid decreased atherogenic lipids and lipoproteins compared to commercial canola oils (Bowen et al., 2019). Thus, high levels of oleic acid in duck skin fat may confer extra value as a food ingredient. In addition, a few reports support the association between oleic acid and flavor. For example, high oleic acid composition provided flavor stability and improved flavor of peanuts (Mugendi et al., 1998; Talcott et al., 2005). The total SFA and USFA contents were significantly different between the groups (p<0.05). Duck skin fat showed the highest total USFA content and the lowest total SFA content, while bovine skin fat exhibited the lowest total USFA and the highest SFA content. More importantly, the ratio of USFA/SFA of duck skin fat was approximately 2-fold higher than that of bovine skin fat (p<0.05). The consumption of unsaturated fat has been linked to the prevention of cardiovascular diseases, such as atherosclerosis (da Silva et al., 2019). Our fatty acid composition analysis demonstrated that duck skin fat contained higher levels of oleic acid and a high USFA/SFA ratio and these might be useful indicators for the increased use of duck skin fat in cooking and food industries.
Quality characteristics of oil and fat are affected by multiple factors, including fatty acid composition, extraction methods, thermal treatment, and the presence of transition metals, pigments, and antioxidants (Choe and Min, 2006). In particular, high levels of polyunsaturated fatty acids may result in the rapid oxidation of fats (Resende et al., 2019). Thus, the oxidative stability of animal skin fats was evaluated during the 90-day storage period at 60°C (Figs. 1A–F). Results of the AV analysis are shown in Fig. 1A. The AV is generated by the hydrolysis and oxidation of triacylglycerol (Ghobadi et al, 2018). The initial AVs (day 0) ranged from 0.64 to 1.81 mg/g fat and the levels were markedly increased and ranged from 3.43 to 6.21 mg/g after 90 days of storage at 60°C. The initial AVs were relatively lower in duck and chicken skin fats than in swine and bovine skin fats. During 90 days of storage, the AVs were increased in all animal skin fats. Specifically, the values were rapidly increased after day 60, probably due to increased hydrolysis and oxidation of triacylglycerol. Increases in AVs also occur in other foods, such as peanuts, during storage. AV levels were increased in raw peanuts stored at room temperature for 720 days (Martin et al., 2018). At the end of the storage period in this study, the AV levels were higher in duck and chicken skin fats than in swine and bovine skin fats. This may have been due to higher concentrations of USFA in the duck and chicken skin fats. USFAs are more susceptible to oxidation and hydrolysis than SFAs (Zhou et al., 2019).
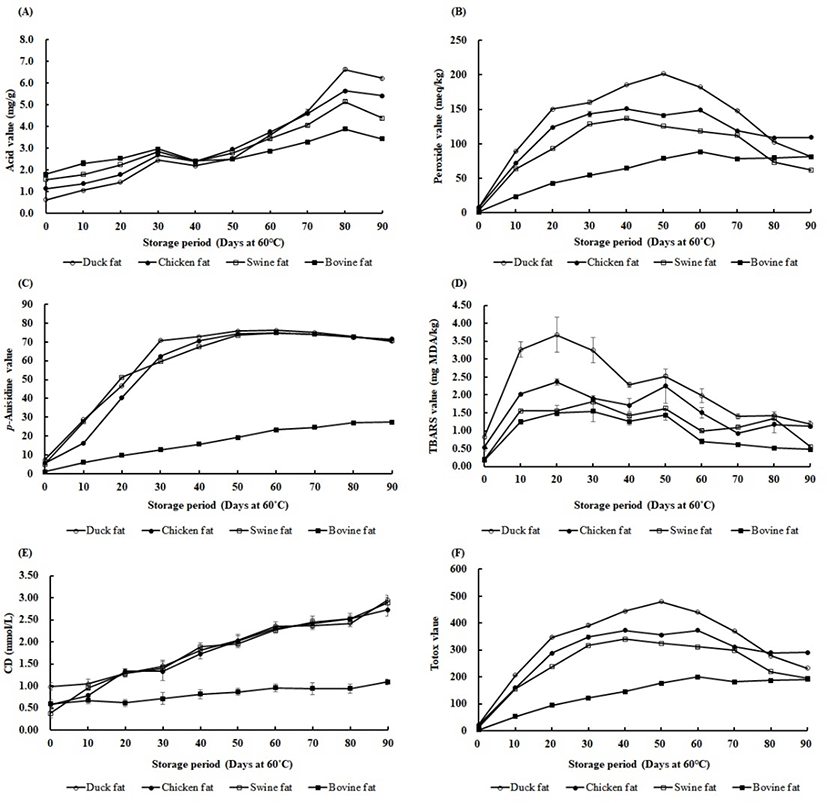
The PV represents the peroxides (primary oxidation products) produced by the oxidation process in fats. Primary oxidation products are broken down into minor substances, such as low molecular weight aldehydes, ketones, alcohols and short-chain hydrocarbons (Kurtys et al., 2016; Strieder et al., 2019). The p-AV indicates the amounts of aldehydes and ketones (secondary oxidation products) and the TBARS value measures the formation of secondary oxidation products, such as malondialdehyde, alkenals, and alkadienals (Shahidi et al., 2003). These measurements have been used to determine the oxidation status of fats and oils.
The data showed a rapid increase in the PVs in duck, chicken, and swine skin fats up to 40 to 50 days of storage (Fig. 1B). After that time, the PVs declined until the end of the storage period. However, in bovine skin fats, the PV increased more gradually and the value was similar to duck skin fats at 90 days (Fig. 1B). This suggests that peroxides were rapidly produced at the beginning of storage in warm conditions (60°C) because of the oxidation of fats. The peroxide product may be further broken down to secondary products, such as aldehydes and ketones (Afonso et al., 2016). This chemical reaction explains the decline in PVs after 40 to 50 days of storage.
The p-AV showed a rapid increase up to 30 to 40 days of storage in duck, chicken, and swine skin fats (Fig. 1C). These p-AVs remained constant until the end of the storage period and there was no significant difference between the three groups at the end of storage (P > 0.05). In contrast, the p-AV in bovine skin fat gradually increased during 60 days of storage (Fig. 1C). The p-AVs tended to depend on the USFA content (p<0.05). High USFA contents have been shown to be the major factor influencing p-AV in samples (Zuo et al., 2017). Our p-AV data also demonstrated a relationship between the amount of USFA and the production of aldehydes and ketones during storage.
Analyzing TBARS is a common technique for measuring lipid peroxidation, particularly the levels of malondialdehyde. In our data, the TBARS value was significantly higher at the beginning of the storage period (at 10 to 40 days) in duck skin fat (Fig. 1D). Chicken skin fat was relatively higher than swine and bovine skin fats (at 10 to 20 days) (Fig. 1D). These results imply that the higher lipid peroxidation in duck and chicken skin fats was due to higher levels of USFA which were more susceptible to oxidation. SFA is less vulnerable to lipid oxidation and that resulted in the lower TBARS value observed in bovine skin fats. Also, higher levels of PV (primary oxidation products) in duck, chicken, and swine fat might have caused the higher TBARS values. In fact, the unstable peroxides can be the result of the decomposition of polyunsaturated fatty acids (Gheisari, 2011). Our data suggest that the addition of antioxidants to duck fats may be useful for improving oxidative stability during storage.
CD is a measure of the oxidative stability of fats or oils where CD is a lipid hydroperoxide formed by triplet oxygen or singlet oxygen (Akhtar et al., 2018). When the oxidation of lipids progresses, the unconjugated double bonds are converted to conjugated structures that absorb ultraviolet radiations of 233 nm (Peri and Saguy, 2015). Our data showed that the CD values gradually increased from the beginning of the storage period in duck, chicken, and swine skin fats (Fig. 1E). The CD value of these skin fats reached the highest point at the end of the storage period. Bovine skin fat, however, remained at low levels during 90 days of storage (Fig. 1E). In fact, a high level of CD values is associated with the presence of higher USFA (Iqbal and Bhanger, 2007). Thus, the observed high CD values for duck skin fat were due to the higher USFA content.
The totox value is known as the sum of 2PV and p-AV. The totox value provides the overall oxidative status of oils and fats (Wai et al., 2009) and lower totox values are expected in better quality cooking oils (Halim et al., 2016). Similar trends of totox values were observed in duck, chicken, and swine skin fats, whereas duck fats showed higher totox values (Fig. 1F). Bovine skin fat showed significantly lower totox values compared to other animal skin fats (p<0.05). In duck, chicken, and swine fats, the totox values continuously increased around 40 to 50 days, then decreased until the end of the storage period. This result has been attributed to the formation of secondary oxidation products which replaced the primary oxidation products (Kasimoglu et al., 2018). These data might also be explained by the ratio of USFA/SFA in the samples. In this regard, a study found that soybean oil containing high amounts of USFA had higher totox values than palm oil with a higher percentage of SFA, such as palmitic acid (Abdulkarim et al., 2007).
Changes in the viscosity of the animal skin fats during the 90-day storage period are presented in Fig. 2. Viscosities increased as the storage time increased in duck, chicken, and swine skin fats (p<0.05). Duck skin fats showed the highest viscosity values, while bovine skin fats showed relatively lower values during the storage period. The formation of high molecular compounds by polymerization during the thermal oxidation process can change the rheological properties (Shin and Kim, 1982). This suggests that polymerized compounds were higher in duck skin fats. Specifically, the increase of viscosity has been explained by the conjugation and isomerization of double bonds in fatty acids and the formation of polymers during thermal oxidation (Ahn et al., 2008). Interestingly, the viscosity of swine skin fat rapidly increased at 90 days. In fact, swine fat contains higher amounts of USFA, particularly linoleic acid (C18:2), than bovine fat. Thus, as shown for the CD (Fig. 1E), the increased viscosity in swine skin fat may be due to the conjugation of double bonds, resulting in polymer formation.
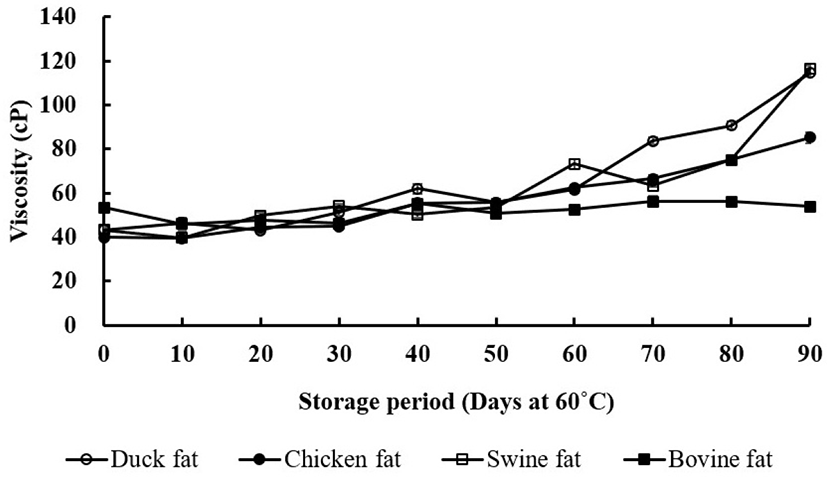
Conclusion
This study evaluated four different animal skin fats (duck, chicken, swine, and bovine fats) extracted with an identical fat extraction method, PHWE. The oxidative stability and quality characteristics of the fats were evaluated under accelerated storage conditions (at 60°C, for 90 days). The data demonstrated that duck skin contained more USFA than other animal skin fats, indicating that duck fat is a healthier edible oil for human consumption. However, duck skin fat was more susceptible to oxidation than other animal skin fats in hot storage conditions. This was probably due to the higher level of USFA in duck skin fats. Supplementation of duck fat with antioxidants might be a reasonable way to prevent oxidation during long-term storage. Further studies on the effects of antioxidant supplementation in duck fat and specific health effects in the human body are needed.