Introduction
The aging process involves the natural degeneration of all the organs of the body over time. In this process, muscular atrophy is an important influencing factor. Muscular atrophy involves the impairment of the major nerves of muscles or a decrease in the absolute volume and strength of muscles under prolonged under certain conditions (Bonewald, 2019; Szentesi et al., 2019).
Muscle cells are involved in the regeneration of muscle fibers containing mechanical, chemical, or degenerative lesions through activation, differentiation, and proliferation (Ceafalan et al., 2014; Karalaki et al., 2009). Differentiation of myoblasts is essential for the formation of muscle fibers involved in the development and regeneration of skeletal muscle (Grefte et al., 2007). These monocyte myoblasts proliferate and differentiate, following which, they fuse with existing muscle fibers to form multinucleated myotubes and myofibers. Proliferation and differentiation of muscle cells occur similarly during development and postnatal birth (Schiaffino et al., 2013). Therefore, promotion of myoblast proliferation and differentiation and induction of myotube hypertrophy should be beneficial for muscle regeneration and control of muscle mass.
Muscle atrophy with aging is accompanied by muscle damage caused by oxidative damage and malnutrition caused by lack of muscle metabolism. Thus, muscle atrophy is triggered by increasing muscle protein degradation and decreased protein synthesis (Zhang et al., 2018). In recent studies, activation of AMP-activated protein kinase (AMPK) increased muscle atrophy F-box (MAFbx)/atrogin-1 and muscle RING finger-1 (MuRF-1) via the transcription factor forkhead box O3a (FoxO3a) (Jaitovich et al., 2015). This process has been reported to be directly involved in muscle atrophy by muscle protein degradation through the activation of the ubiquitin-proteasome pathway (Jaitovich et al., 2015; Nakashima and Yakabe, 2007; Tong et al., 2009). Since muscle mass represents a balance between muscle cell replication, protein synthesis, muscle cell death and protein degradation (Scicchitano et al., 2018), increased muscle mass can prevent muscle atrophy by causing an increase in muscle differentiation and inhibiting muscle loss.
Deer antler (Cervi parvum Cornu) is a non-osteolytic horn of Cervus nippon Temminck or Marcus (Cervus elaphus L) and has long been used in oriental countries, such as China and Korea. Deer antler has been reported to contain hexose, pentose, uronic acid, sialic acid, free amino acids, minerals, prostaglandins, and gangliosides in glycolipids (Jhon et al., 1999; Sunwoo et al., 1995). Water-soluble proteins, polypeptides, and free amino acids have been shown to be the main biologically active components of deer antler (Moreau et al., 2004). In addition, there is evidence that deer antler extract has strong potential for promoting bone growth and development (Chen et al., 2015; Shi et al., 2010). Additionally, Chen et al. (2014) estimated through microarray analysis that the anti-fatigue effect of collagen and protein from deer antler extracts is mediated by the increased expression of genes involved in muscle contraction and development.
By evaluating the effect of strengthening muscle differentiation and inhibiting muscle atrophy by antler extract having various activities, it was attempted to investigate the possibility of application as a functional material to suppress muscle atrophy due to aging. Therefore, the effects of deer antler extract on the promotion of myoblast differentiation and 5-aminoimidazole-4-carboxamide ribonucleoside (AICAR)-induced muscle atrophy were examined using C2C12 fibroblast.
Materials and Methods
Dulbecco’s modified Eagle’s medium (DMEM), fetal bovine serum (FBS), horse serum (HS), and penicillin/streptomycin (PS) were purchased from WELGENE (Daegu, Korea) and were used for cell culture and differentiation. AICAR and 3-(4,5-dimethylthiazol-2-yl)-2,5-diphenyl tetrazolium bromide (MTT) were obtained from Sigma-Aldrich Chemical (St. Louis, MO, USA). Dried antler (Cervus canadensis) from adult male Korean elk was obtained from Kwang Dong Pharmaceutical (Seoul, Korea). According to the previous report, antler water extract (HWE) and fermented antler (FE) were prepared. The antler powder was extracted by refluxing for 3 h at 95°C after adding 8 times the amount of water. Fermented antler was extracted by adding 8 times the amount of phosphate buffer (PBS, 50 mM, pH 6.0) the antler powder, fermenting it with Bacillus subtilis for 48 h, and refluxing for 3 h at 95°C. The ultrasonic extract (UE) was extracted by adding 8 times the amount of PBS to the antler powder for 30 minutes at 120 kHz ultrasonic waves (AUG-R3-900, Asia Ultrasonic, Bucheon, Korea). After ultrasonic extraction, reflux extraction was performed at 95°C to obtain an UE. After adding 8 times the amount of PBS (50 mM, pH 7.8) to the antler powder and suspending it, Alcalase equivalent to 0.5% of the antler powder was added, followed by hydrolysis at 50°C for 8 h. After hydrolysis, reflux extraction was performed at 95°C to obtain an antler enzyme hydrolysate (ET). The antler extracts were concentrated and lyophilized to be used in the further experiment.
According to the Sunwoo et al. (1998) method, the collagen content was calculated by multiplying the content of hydroxyproline by 7. According to the method of Stegemann and Stalder (1967), the content of hydroxyproline before and after acid hydrolysis with 6 N HCl was measured using HPLC. A Phenomenex Luna C18 Column (250×4.6 mm, 5 μm) was employed, using following mobile phase: sodium acetate buffer (pH 4.3) containing 3% glacial acetic acid and acetonitrile were mixed with 650 and 350 mL, respectively. The flow rate was 1 mL/min, hydroxyproline was identified at a wavelength of excitation 260/emission 330 nm, and the injection quantity was 20 μL. The collagen content was calculated by multiplying the difference by 7 to the hydroxyproline content before and after acid hydrolysis.
The myoblast cell line C2C12 used in this study was purchased from the American Type Culture Collection (Manassas, VA, USA). C2C12 myoblasts were maintained at 37°C and 5% CO2 using a growth medium containing 90% DMEM, 10% FBS, and 100 unit/mL PS. To maintain cells at an appropriate density, they were passaged every 48 h. Differentiation medium containing 90% DMEM, 10% HS, and 100 unit/mL PS was used to differentiate C2C12 myoblasts into myotubes. To confirm the morphological changes during the differentiation process, an inverted microscope (Inverted microscope, Carl Zeiss, Gottingen, Germany) was used to observe cells at a magnification of 200×.
The cell viability of C2C12 cells was measured using an MTT assay. C2C12 cells were seeded into a 96-well plate at a concentration of 1×106 cells/mL the day before the experiment. Deer antler extracts (0–1,000 μg/mL) were incubated with cells for 24 h. Subsequently, the culture supernatant was discarded and 5 mg/mL MTT dissolved in DMEM was added to each well; the plate was then covered with aluminum foil to create dark conditions and incubated for 2 h. After removing the MTT solution, 100 μL of dimethyl sulfoxide was added to the cells and left for 2 h. The absorbance was measured at 540 nm using a microplate reader (Molecular Devices, Sunnyvale, CA, USA).
Myotube length and diameter were measured using images obtained through Jenner-Giemsa staining of C2C12 differentiated cells (Veliça and Bunce, 2011). The cultured cells were washed with PBS, fixed with 100% methanol for 5 min, and air dried for 10 min. The Jenner staining solution (Sigma-Aldrich, St. Louis, MO) was diluted 3-fold with 1 mM sodium PBS (pH 5.6); 1 mL of this solution was incubated in the wells for 5 min and then the cells were washed with distilled water. The cells were then incubated for 10 min at 25°C with 1 mL Giemsa stain diluted 1:20 with 1 mM sodium PBS (pH 5.6) and then washed again with water. Cells in each well were observed using a MM-400 microscope (Nikon, Tokyo, Japan) and photographed in nine randomly selected areas using a digital microscope camera JENOPTIK ProgRes speed Xtcore 3 and the ProgRes Image Capture Software (Jenoptik Optical Systems GmbH, Jena, Germany). Myotube length and diameter were measured and quantified using the Image-J software (Scion, Frederick, MD, USA).
Total RNA from C2C12 cells was extracted using the TRIzol® reagent (Invitrogen, CA, USA), according to the manufacturer’s protocol. Quality controlled RNA samples with high optical density ratios were treated with RQ1 RNase-free DNase I (Promega, WI, USA). One microgram of total RNA was reverse transcribed using SuperScript® III Reverse Transcriptase (Invitrogen) with oligo d (T) primers. qRT-PCR was performed using the Taqman Gene Expression Master Mix (Applied Biosystems, Foster City, CA, USA), and quantitative analyses were conducted using the StepOne plus Software V. 2.0 (Applied Biosystems). All results were normalized against the expression of the control gene, GAPDH (NM_008084.2), using the ΔΔCt method (Livak and Schmittgen, 2001). The information of primers for the qRT-PCR of tested genes related to muscle differentiation and atrophy were as follows: myogenin differentiation 1 (MyoD1) (NM_010866.2), myogenic factor 5 (Myf5) (NM_008656.5), AMPK (NM_001013367.3), forkhead box O3 (FoxO3a) (NM_019740.2), muscle RING-finger protein 1 (MuRF-1) (NM_001039048.2), and myogenin (NM_031189.2).
All experimental results were obtained using the SPSS ver. 18.0 (SPSS, Chicago, IL, USA) statistical program. Data represent the mean±SD. Differences among the groups were evaluated by one-way analysis of variance (ANOVA) and Tukey’s multiple test. Student’s t-test was used to analyze differences between groups with normally distributed data.
Results and Discussion
The collagen content of HWE, ET, UE, and FE, it was 20.09±0.19, 29.07±0.12, 27.32±0.09, 23.58±0.11 μg/mg of extract, respectively, and it was confirmed that the collagen content of the extract increased due to fermentation, enzyme, and ultrasonication treatment (Table 1). In particular, the content of hydroxyproline (4.15±0.02 μg/mg of extract) and protein (295.05±11.22 mg/g of extract) as well as collagen in ET was higher than that of other antler extracts.
Deer antler extracts, including HWE, FE, ET, and UE were used for the evaluation of myoblast C2C12 cells differentiation activity. These extracts had protein contents of 225.1–295.1 mg/g extract (data not shown). Upon evaluation of the cytotoxicity of the antler extracts against C2C12 cells (Fig. 1), all extracts except the UE did not show a cytotoxicity of up to 1,000 μg/mL. UE-treated cells showed a cell viability of 90.7%–88.9% upon treatment with 200–1,000 mg/mL extract. Deer antler extracts were found to have no or low cytotoxicity at 1,000 μg/mL. Therefore, further experiments were conducted using up to 200 μg/mL of extracts, with little cytotoxicity.
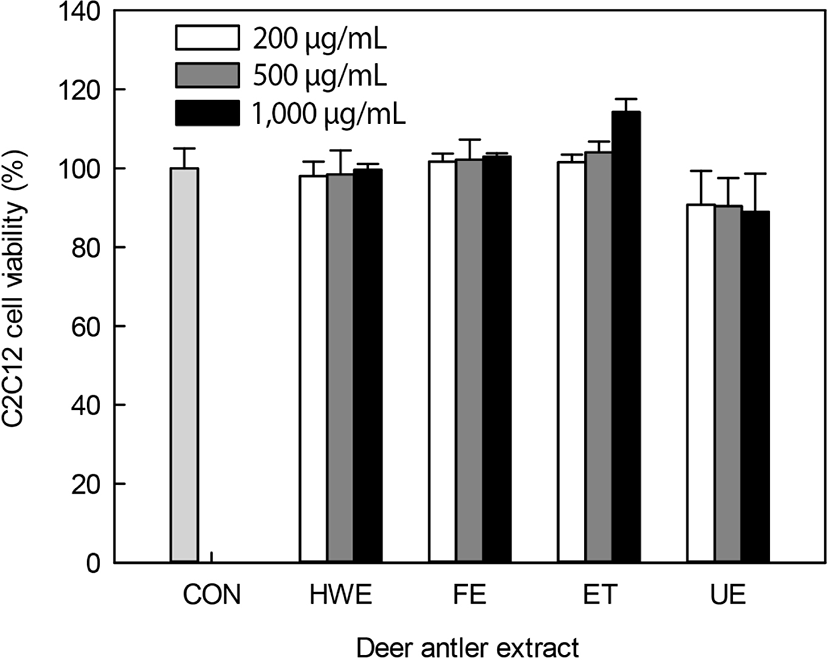
Deer antler extracts have been widely used in traditional Chinese medicine for centuries and are generally believed to provide nourishment, strengthen the kidneys, strengthen the spleen, strengthen bones and muscles, and promote blood flow (Wu et al., 2013). Previous studies have reported that the collagen and protein components of deer antler extracts are major bioactive substances with effects, such as anti-fatigue, anti-stress (Fengyan et al., 2001), and bone growth effects (Niu et al., 2012) and stimulation of hematopoietic function (Lee et al., 2012).Kitakaze et al. (2016) reported that collagen hydrolysates promoted differentiation and increased size of myoblast C2C12 cells. Hydroxyprolyl-glycine, a collagen hydrolysate, has been reported to induces myogenic differentiation and root canal hypertrophy by increasing the size of the root canal and the expression of root canal specific myosin heavy chain (MyHC) and tropomyosin structural proteins. Hyp-Gly presumably promotes myogenic differentiation by activating the PI3K/Akt/mTOR signaling pathway according to peptide/histidine transporter 1 for entry into cells (Kitakaze et al., 2016; Li et al., 2016; Niu et al., 2012).
To determine the degree of myotube differentiation during C2C12 cells differentiation, Jenner-Giemsa staining was performed on the second and fourth days of C2C12 cells differentiation to measure changes in myotube morphology, such as myotube length and diameter (Fig. 2 and 3).
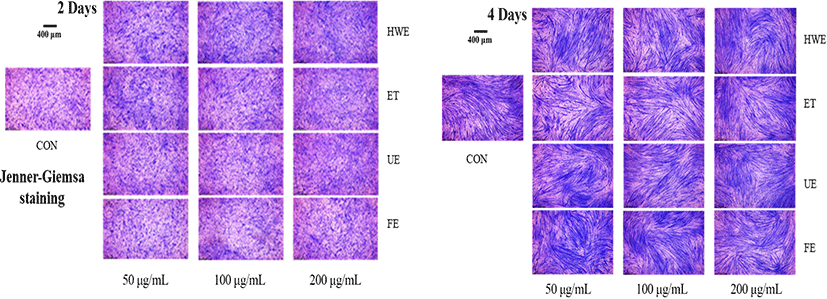
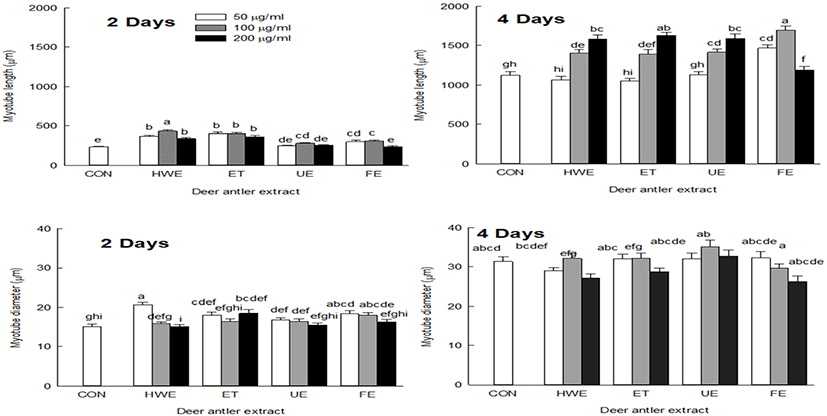
On the second day of cell differentiation, myotube length was increased in cells treated with deer antler extracts compared with the control group (CON). On the fourth day, myotube length was increased in cells treated with all deer antler extracts except for FE. The cells treated with FE showed increased myotube length at extract concentrations of 50 and 100 μg/mL and shortened myotube length when treated with extract concentration of 200 μg/mL (Fig. 3).
On day two of cell differentiation, cells treated with deer antler extract showed a similar diameter to that of CON, but on day four of cell differentiation, cells showed a tendency to have increased myotube diameter compared to CON (Fig. 3). Upon treatment of cells with deer antler extract, myotube length was increased by promoting the myotube differentiation capacity, and the myotube diameter also tended to increase with increasing myotube length.
In the differentiation phase, the length of myoblasts become increased, fused with localized cells and converted into tubular multinuclear cells. When differentiation is induced from myoblasts to myotubes, the number of muscle fibers increases due to the fusion between cells and the thickening of muscle fibers also occurs (Bentzinger et al., 2012; Burattini et al., 2004). Net protein balance affects the size of individual myotubes and myofibers, where an increase in net protein levels leads to muscle hypertrophy (White et al., 2010) and contributes to an increase in skeletal muscle mass (Ontell et al., 1984).
Muscle differentiation is regulated by myogenic regulatory factors (MRFs), such as MyoD1 and Myf5 (Braun et al., 1992; Hyatt et al., 2003). The effect of the antler extract on the expression of muscle differentiation factors MyoD1 and Myf5 was measured on days two and four of C2C12 cell differentiation, respectively (Fig. 4). The expression levels of MyoD1 were not significantly affected by myoblast differentiation on days two and four, whereas Myf5 expression levels were high on days two and four compared with the CON. Deer antler extracts significantly increased the expression level of Myf5 (p<0.05). In particular, it was found that the level of Myf5 increased in a concentration-dependent manner when the HWE was added on day four of myogenic differentiation. However, increasing concentrations of UE and FE tended to decrease the expression level of Myf5. These results indicated that antler extract was not only involved in increasing the size (length and diameter) of myotubes, but also in increasing the expression level of myogenic differentiation factor that promotes myoblast C2C12 cell differentiation.
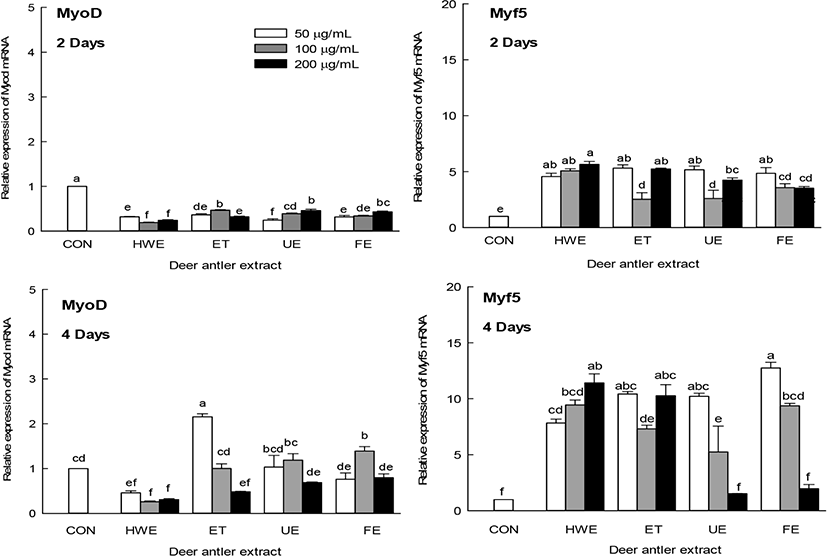
Muscle differentiation is regulated mainly by MRFs, such as MyoD1 and Myf5, which are involved in establishing myogenic lineages (Braun et al., 1992). Later in muscle differentiation, there is an increase in the expression of MyHC, a major structural protein of myotubes (Soundharrajan et al., 2019). Deer antler extracts were also found to be involved in the increase in the expression levels of muscle differentiation factors (Fig. 4), which may increase the size of the myotubes (Fig. 3).
As a result of a 0.25–2 mM treatment of AICAR to create a muscle atrophy model in myoblast cells, AMPK expression increased in an AICAR concentration-dependent manner (Fig. 5). C2C12 cells showed no cytotoxicity when treated with 2 mM of AICAR (data not shown). Therefore, C2C12 cells were treated with 2 mM AICAR to induce muscle atrophy, and the effect of the inhibition of muscle atrophy through the addition of antler extracts was confirmed.
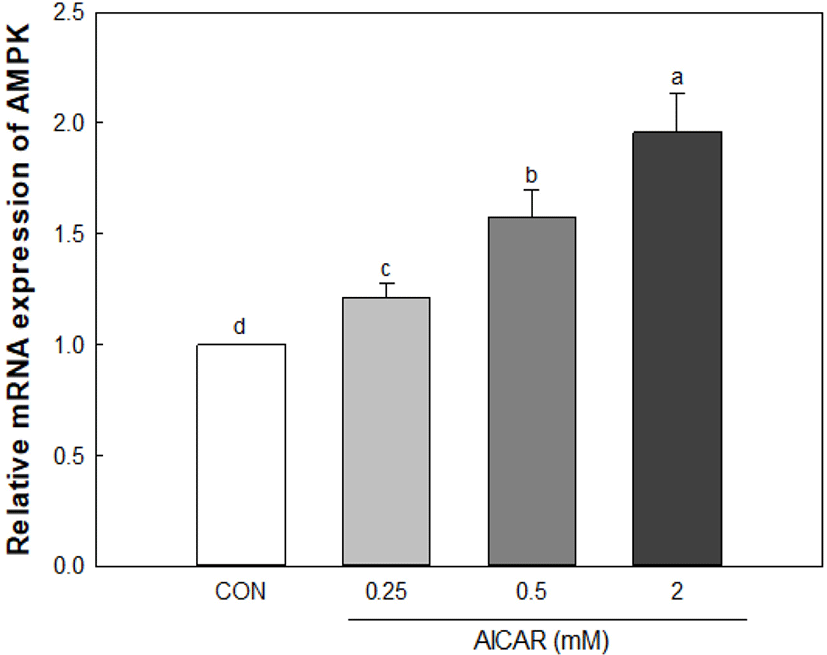
The effects of deer antler extracts (50 and 200 μg/mL) on the expression levels of muscle atrophy factors (FoxO3a and MuRF-1) were measured in AICAR-induced muscle-atrophy cells (Fig. 6). Upon AICAR treatment, the expression of AMPK increased in C2C12 cells and deer antler extract-treated muscle-atrophy cells compared to the CON. The expression levels of AMPK in deer antler extract-treated cells were higher than those in cells treated with AICAR alone (CON group). The muscle atrophy factors FoxO3a and MuRF-1 showed significantly higher expression levels in the AICAR treated group (CON) than in the normal group (NOR). In deer antler extract-treated muscle-atrophied cells, the increase in the expression level of the muscle atrophy factor MuRF-1 tended to be lower than that in the CON group cells. In particular, the expression level of FoxO3a in deer antler extract-treated atrophied cells was significantly lower than that in cells treated with AICAR alone (p<0.05).
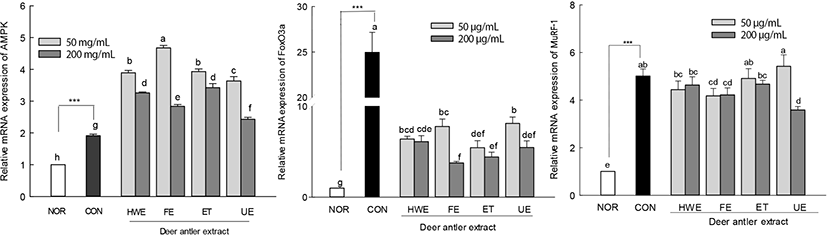
Recent studies have shown that muscle atrophy occurs due to increased proteolysis due to the activation of the ubiquitin-proteasome pathway (Bodine and Baehr, 2014; Jagoe and Goldberg, 2001; Liu et al., 2016). In particular, muscle atrophy F-box (MAFbx)/atrogin-1 and MuRF-1, which are muscle-specific ubiquitin ligases, are expressed early in the muscle atrophy process and are directly involved in muscle protein degradation (Bodine and Baehr, 2014; Liu et al., 2016). In addition, when muscle atrophy is induced, expression of genes, such as myogenin and MyoD, muscle-specific transcription factors involved in myogenic differentiation, is reduced by activating muscle-specific gene expression (Hyatt et al., 2003; Tintignac et al., 2005).
The expression levels of MyoD1 and myogenin, which are muscle differentiation factors, were lower than those of normal cells upon treatment with AICAR alone (Fig. 7). In deer antler extract-treated muscle-atrophied cells, the expression levels of muscle differentiation factors MyoD1 and myogenin were higher than those in cells treated with AICAR alone. The expression levels of MyoD1 in the enzyme-treated deer antler extract (ET, 200 μg/mL)-treated muscle-atrophied cells were significantly higher than those of normal cells (p<0.05). In addition, the expression levels of myogenin in the enzyme-treated deer antler extract (ET, 50 and 200 μg/mL) and fermented deer antler extract (FE, 200 μg/mL)-treated muscle-atrophied cells were significantly higher than that in normal cells. The treatment of AICAR-induced atrophied cells with deer antler extract inhibited the expression of the muscle atrophy factors FoxO3a and MuRF1. In addition, deer antler extract treatment restored the expression levels of myoD1 and myogenin to the same or higher levels as those of normal cells.
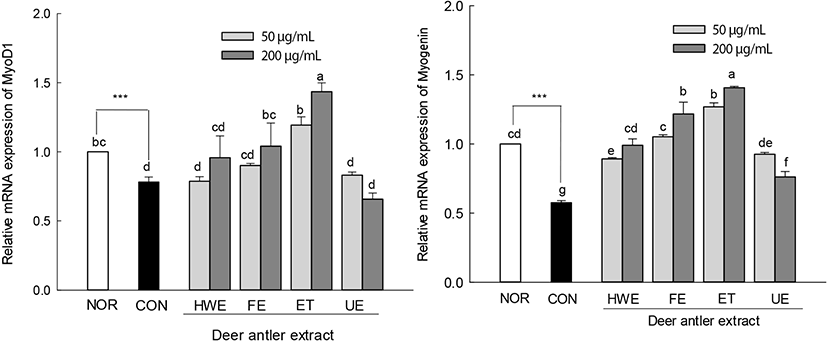
In Fig. 6, muscle atrophy factors FoxO3a and MuRF-1 can be seen to be increased upon AICAR treatment, and their increase results in muscle atrophy. In addition, in Fig. 7, the expression levels of MyoD and myogenin, which are myogenic factors, can be seen to decrease upon AICAR treatment. However, MuRF-1 expression decreased upon treatment with antler extract, and the expression factor of myogenesis markers increased. Deer antler extracts seemed to contribute more to the inhibition of muscle atrophy by increasing the expression of muscle differentiation factors than by decreasing the expression of muscle atrophy factors.
The increase in skeletal muscle mass occurs due to an increase in muscle protein synthesis (MPS) compared to muscle protein breakdown (Rennie, 2007). To increase skeletal muscle or inhibit muscle loss, it is important to have both an increase in myogenic capacity and an inhibition of muscle loss. Therefore, the supply of high-quality dietary protein, which is an essential nutrient for promoting muscle and overall metabolic health, is important (Arentson-Lantz et al., 2015). Deer horn extract is an excellent source of protein that is especially rich in collagen type I (Li et al., 2016). Therefore, the active substances of antler extract to myogenesis and inhibit the muscle atrophy are presumed to be protein, collagen and hydroxyproline. It has also been reported that collagen breakdown products, which form a major component of antlers, are involved in the activation of mammalian target of rapamycin (mTORC1) and MPS. The mechanistic/mTORC1 pathway is the central molecular pathway of MPS and is activated by a variety of stimuli, including, but not limited to, resistance exercise (Bolster et al., 2003), insulin (Conejo et al., 2001), and dietary amino acids (Gordon et al., 2013). We found four distinct effects of treating C2C12 cells with antler extract: 1) increase in myotube size, 2) increase in muscle differentiation factor (MyoD1 and Myf5) expression, 3) suppression of muscular atrophy factor (FoxO3a and MuRF-1) expression, and 4) increase in the expression of the muscle differentiation factors (MyoD1 and myogenin), in a muscle atrophy model.
Conclusion
Several studies have reported that collagen, a major component of deer antler, helps myogenesis. In this study, experimental results on C2C12 cells suggested that antler extract has the ability to inhibit muscle atrophy and promote muscle differentiation by increasing the expression of myoblast differentiation factors MyoD, Myf5 and myogenin. Further experiments will be conducted to identify muscle differentiation promoting substances.