Introduction
Pesticides are chemical or biological substances that inhibit the growth of living organisms or prevent and destroy pests for improving product yields. The effects of pesticide exposure include respiratory, neurological, gastrointestinal, and skin problems (Azaroff and Neas, 1999; Hoppin et al., 2002; Salameh et al., 2006). Pesticides cause biochemical changes, leading to clinical health signs (Balani et al., 2011; Jonnalagadda et al., 2010). These biochemical changes result from the destructive and degenerative effects of pesticides on the organs (Khan et al., 2013; Mossalam et al., 2011). Farm workers exposed to pesticides were found to have significantly increased serum concentrations of urea and creatinine (CREA) (Haghighizadeh et al., 2015; Ritu et al., 2013). Pesticides are used globally for improving yields and the quality of agricultural products (Eddleston et al., 2008; Songa and Okonkwo, 2016; Yan et al., 2018). However, the abuse of pesticides has led to food and environmental contamination (Carvalho, 2017; Li et al., 2019; Liu et al., 2016).
Pesticides are effective to increasing agricultural yields, but it is not easy to management and monitoring (Khan et al., 2008; Peshin and Zhang, 2014; Peshin et al., 2009). For increasing crop yield and quality, pesticides are classified according to their purpose, such as herbicides, pesticides, and fungicides. Pesticides can also be classified according to the pest's origin or structure or activity site, such as fungicides, fumigants, herbicides, and insecticides (EPA, 2020). Therefore, global regions (e.g., Codex, EU, US, Canada, India, Australia) have established policies on maximum residual limits in food and feedstuff to limit pesticide residues in human and animals (Handford et al., 2015).
Triazole fungicides (e.g., flutriafol, propiconazole, tebuconazole, and tetraconazole) are used on different types of plants to protect against different fungal diseases (Lass-Flörl, 2011). Among these fungicides, flutriafol [(R,S)-1-(2-fluorophenyl)-1-(4-fluorophenyl)-2-(1H-1,2,4-triazol-1-yl) ethanol] is commonly used to control leaf and ear diseases in cereal crop and in seed treatment (FAO, 2011). It is a chiral triazole fungicide employed to control plant pathogens. The fungicidal mechanism of such pesticides inhibits ergosterol biosynthesis and cell wall synthesis (Song et al., 2019; Yang et al., 2020).
Several studies have found that fibrosis is caused by pesticide exposure. Exposure to ethylated dialkylphosphates, which are known to have immunomodulatory potential, can induce long-term damage to the heart, leading to fibrosis (Medina-Buelvas et al., 2019). Exposure to residual pesticides increased liver fibrosis and nonalcoholic fatty liver disease in HepG2 cells and rat liver (Kwon et al., 2021a; Kwon et al., 2021b). Furthermore, meta-analysis revealed that the risk of idiopathic pulmonary fibrosis increased in agricultural workers exposed to pesticides (Park et al., 2021; Ramani et al., 2021). Pesticide residues have a substantial influence on growth and health of livestock and humans (Abou-donai, 2003; Cordes and Foster, 1988; Moses, 1989). Although flutriafol has been detected in cells (e.g., HepG2, Neuro2A, NIH/3T3, SH-SY5Y, VERO), humans, and laboratory animals, studies on pig meat and related products are scarce. Therefore, this study investigated the potential effects of flutriafol residues in pork and related products.
Materials and Methods
All experimental procedures were reviewed and approved by the Institutional Animal Care and Use Committee of the National Institute of Animal Science, Korea (No. 2019-1576).
Pigs were purchased from the Darby (Anseong, Korea). Twenty castrated male pigs (Landrace×Yorkshire, 72.0±2.2 kg) were housed in individual pens (2.1×1.4 m). For the experimental period including acclimatization, the housing conditions were: a light-dark cycle of 12:12 h and a constant temperature (22±2°C) and relative humidity (55±5%). The pigs were divided into six groups according to acceptable daily intake on OECD test guideline 505: control (n=3), T1 (0.313 mg/kg bw/d; n=3), T2 (0.625 mg/kg bw/d; n=3), T3 (3.125 mg/kg bw/d; n=3), T4 (6.25 mg/kg bw/d; n=4), and T5 (12.5 mg/kg bw/d; n=4). Animals were fed according to the Korean feeding standards for pig (2017). Flutriafol (NH chemical, Ulsan, Korea) was thoroughly mixed into the feed according to the concentrations per body weight. Animals were treated a diet exposed to flutriafol twice daily for 28 d. At the end of the experimental period, all pigs were anesthetized with the T61 agent. After exsanguination, the blood, liver, kidney, ileum, muscle, and fat tissues were quickly removed. These tissues were immediately frozen in liquid nitrogen for residue analysis and then stored at −80°C. Some tissues were fixed with 10% neutral buffered formalin (NBF; Sigma-Aldrich, St. Louis, MO, USA) for histological analysis. Average daily weight gain (ADG), average daily feed intake (ADFI), and feed conversion ratio (FCR) were calculated as follows: ADG = (finish weight – start weight) / age (days), ADFI = provide feed amount - residual feed amount, FCR = feed intake / average daily gain.
Blood samples were collected with a suitable vacutainer tube containing no anticoagulants. The serum was extracted using centrifugation (700×g for 15 min at 4°C) and then kept at −80°C. A total of 15 parameters, consisting of glucose (GLU), CREA, blood urea nitrogen (BUN), phosphate (PHOS), calcium (CA), total protein (TP), albumin globulin (ALB), alanine aminotransferase (ALT), alkalinephosphate (ALKP), gamma glutamyl transpeptidase (GGT), total bilirubin (TBIL), cholesterol (CHOL), amylase (AMYL), and lipase (LIPA) were determined using the VetTest chemistry analyzer (IDEXX, Westbrook, ME, USA), following the manufacturer’s procedure.
To quantify flutriafol, the collected samples (2.0 g tissue or 2.0 mL blood) were mixed with distilled water (10 mL), set for 10 min, and mixed with acetonitrile and sodium chloride (20 mL and 5 g, respectively). The samples were stirred using a vortex for 10 s and shaken for 60 min. The extract was centrifuged at 3,500×g for 5 min. Primary-secondary amine (PSA) and octadecylsilane (C18) were used for analyzing the samples. The filtered samples were injected and the peak area was compared to estimate the residue levels. The samples (5 μL each of plasma, liver, kidney, muscle, and fat) were injected into a liquid chromatography-tandem mass spectrometer (LC-MS/MS). The quantitative limit of the assay was 0.01 mg/kg. Residue analysis was conducted by an ExionLC system with a QTRAP 4500 mass spectrometer (SCIEX, Framingham, MA, USA). The conditions were: columns (100×2.0 mm, 3.0 μm) maintained at 40°C, mobile phase composition of 10 mM ammonium acetate and methanol, linear gradient mode from 20% to 90% methanol, and flow rate of 0.1 mL/min.
The tissue (i.e., liver, kidney, muscle, fat, and ileum) samples were fixed with 10% NBF and then dehydrated (from 70% to 100% EtOH), embedded, cut (5 μm-thick), mounted, and heated (40°C) for 1 h on a hot plate. For staining, the sections were dewaxed with xylene, rehydrated (from 100% to 70% EtOH), and washed with distilled water. The sections were stained using Masson’s trichrome (MT) staining reagents following the manufacturer’s protocol. The slices were observed under 100× magnification in an optical microscope.
All results including growth performance and biochemical analysis were analyzed using one-way ANOVA (Prism 5.01, GraphPad Software, San Diego, CA, USA), followed by Tukey’s multiple comparison post-hoc test. Results are showed as mean and SEM. A p-value of less than 0.05 between the control and treatment groups was considered to be significant.
Results and Discussion
The growth performances of flutriafol-exposed pigs were not significantly different between control and treatment groups (data not shown). Briefly, the difference in initial (72.0±2.36 kg) and final (96.9±4.48 kg) body weights was not statistically significant. Furthermore, no significant differences were detected in ADG, ADFI, and FCR of flutriafol treated pigs as compared to control, despite its acute toxicity. Body weight of flutriafol treated rats was increased compared to control at 1 and 2 wk. However, the body weights at 3 wk were not significantly different (Kwon et al., 2021a). No significant effects of tebuconazole treatment were observed on body condition, growth, and sex ratio of chicks (Lopez-Antia et al., 2021). In this study, flutriafol exposure did not affect the growth performances in pigs, similar to the observations of previous studies.
Table 1 presents the effect of pesticide exposure on biochemical properties of pig serum. The parameters are as follows: GLU, CREA, BUN, PHOS, CA, TP (TP = ALB + GLOB), ALB, ALT, ALKP, GGT, TBIL, CHOL, AMYL, LIPA. CREA, BUN, BUN/CREA ratio, TP, ALB, GLOB, ALB/GLOB ratio, ALT, GGT, AMYL, and LIPA showed significant differences from those of the control (p<0.05). In particular, CREA decreased significantly in the T4 and T5 treatment groups compared with control (p<0.05). BUN, ALT, and LIPA showed a significant increase in treatment groups than that of control (p<0.05). No significant differences were detected in the other biochemical parameters (i.e., GLU, PHOS, CA, ALKP, TBIL, and CHOL). The principal component analysis did not show a difference between control and treatment groups (data not shown).
Reference ranges: GLU (85–160), CREA (0.5–2.1), BUN (6–30), PHOS (3.6–9.2), CA (6.5–11.4), TP (6.0–8.0), ALB (1.8–3.3), GLOB (2.5–4.5), ALT (9–43), ALKP (92–294), GGT (16–30), TBIL (0.1–0.3), CHOL (18–79), AMYL (271–1,198), LIPA (10–44).
GLU, glucose; CREA, creatinine; BUN, blood urea nitrogen; PHOS, phosphate; CA, calcium; TP, total protein (TP=ALB+GLOB); ALB, albumin globulin; GLOB, globulin; ALT, alanine aminotransferase; ALKP, alkalinephosphatase; GGT, gamma glutamyl transpeptidase; TBIL, total bilirubin; CHOL, cholesterol; AMYL, amylase; LIPA, lipase.
Blood biochemistry values provides important biological information to humans and animals. The results of our study showed that pesticide exposure affects pigs, resulting in significant differences in parameters such as CREA, BUN, ALT, and LIPA. These biochemical changes can lead to destructive and degenerative changes in the renal corpuscles based on pesticides (Khan et al., 2013; Mossalam et al., 2011). Farmers exposed to pesticides had significantly higher serum levels of urea and CREA (Haghighizadeh et al., 2015; Ritu et al., 2013). The urea and CREA levels showed significant differences between control and treatment groups. However, CREA levels in the T5 group were lower than those in control. Urea is formed by ammonia produced in the liver and is excreted through the kidney. Urea and CREA excreted by the kidneys are used as biomarkers to determine kidney damage (Singh et al., 2011). Organophosphates are widely used pesticides. The organophosphate pesticides increase CREA levels because of impaired glomerular function and damage to the renal tubules (Mohssen, 2001). ALT, also known as transaminases, provide important information about damaged hepatocytes (Hernandez et al., 2013). The ALT levels caused by pesticide-induced stress are associated with the production of reactive oxygen species and oxidative tissue damage (Patil et al., 2009, Singh et al., 2011). In particular, increase in blood GLU, insulin, triglycerides, and LIPAs exposed to organophosphorus pesticides have been reported in several studies (Gangemi et al., 2016; Kamath and Rajini, 2007; Rodrigues et al., 1986; Romero-Navarro et al., 2006). Immobilized LIPA is used as a biosensor to determine TG due to its accuracy and efficiency (Chandra et al., 2020; Escamilla-Mejía et al., 2014). Significant elevations were observed in urea and CREA concentrations of serum samples exposed to pesticides. These elevations correspond to renal impairment and renal dysfunction (Pandya et al., 2016). Serum CREA and urea, known as renal biochemical markers, were significantly different between control and treatment groups. The elevated serum urea observed in response to pesticide exposure in this study could be explained by impaired synthesis and protein metabolism due to hepatic dysfunction. Together, CREA, BUN, ALT, and LIPA can act as potential biomarkers to detect exposure to flutriafol.
The linear and quadratic equations of flutriafol exposure concentrations were used for determining maximum residue limits in liver (Fig. 1A), kidney (Fig. 1B), fat (Fig. 1C), muscle (Fig. 1D), and blood (Fig. 1E). The residual levels of all tissues increased with an increase in flutriafol concentration. The quadratic equations for liver (r2=0.9982, p<0.001), kidney (r2=0.9960, p<0.01), muscle (r2=0.9928, p<0.05), and blood (r2=0.9856, p<0.01) showed significant differences between control and treatment groups (Fig. 1). The linear equations of liver (r2=0.9951), kidney (r2=0.9599), muscle (r2=0.9092), and blood (r2=0.9847) were concentration dependent (Fig. 1B–D). However, although the residual levels increased according to treated flutriafol concentrations in fat, there was no significant differences between the treatment groups (Fig. 1C).
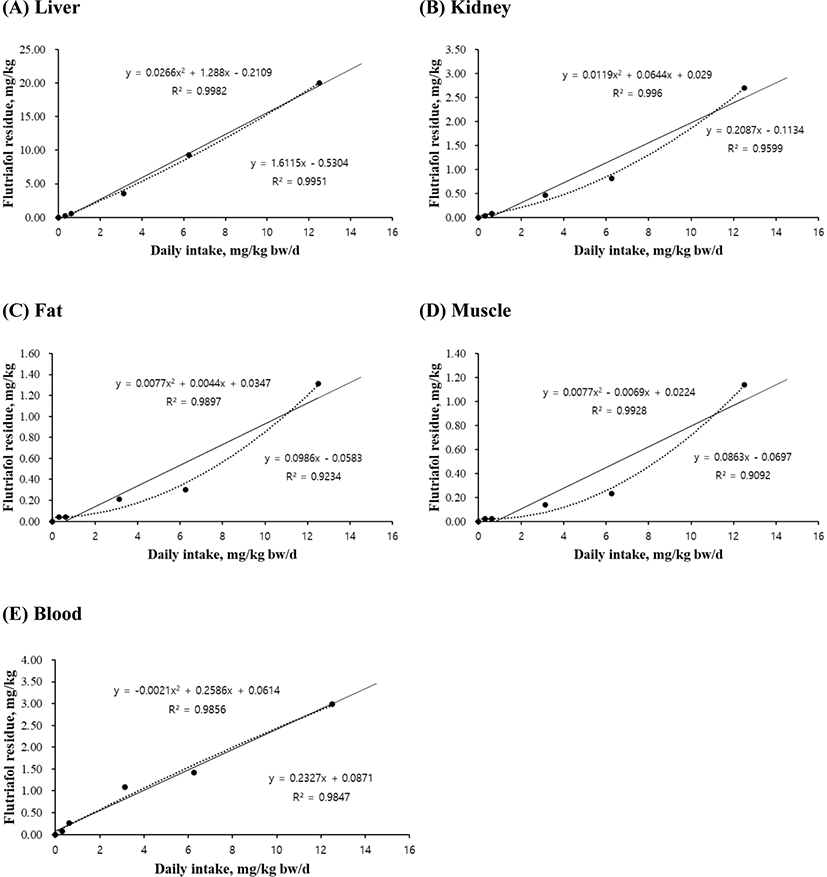
Flutriafol, known as conazole fungicide, is used to control leaf and ear diseases in cereals (FAO, 2011) and to prevent fungal diseases (Bhuiyan et al., 2015; Lass-Flörl, 2011). Exposure to pesticides through oral, dermal, or inhalation routes is associated with low or moderate toxicity (Kwon et al., 2021b; Shahinasi et al., 2017; Toumi et al., 2017). The high performance liquid chromatography method was used to establish the maximum residue limits of flutriafol exposure in wheat and soil (Yu et al., 2012; Zhang et al., 2014). Therefore, our results suggest that the residual values for different tissues showed variations according to pesticide concentrations. Taken together, the equations for graded levels of flutriafol will help predict the risk assessment and maximum residue limits in pig production and meat safety.
In this study, the histological changes in liver, kidney, muscle, fat, and ileum tissues of pig due to exposure to flutriafol were assessed using MT staining (Fig. 2). Fibrosis deposition and tissue destruction at different flutriafol concentrations were observed for all treatment groups. Fibrosis in treatment groups was measured by MT staining on the portal areas and lobular boundary of the liver. The glomeruli, tubules, and vasculature in kidney tissues were stained blue. Kidneys showed interstitial fibrosis and glomerulosclerosis at different flutriafol concentrations. Villus and lamina propria in ileum were deteriorated and exhibited prominent blue staining after flutriafol exposure as compared to that in control. Muscle and fat tissues were stained with blue after being exposed to flutriafol. Collagen fibrosis also showed concentration-dependent effects in the treatment groups compared to control. The ethylated dialkylphosphates, known as metabolites of organophosphorus pesticides, are known to aggravate heart fibrosis and inflammation (Medina-Buelvas et al., 2019). In our study, the flutriafol also showed changes in vacuoles of the proximal tubules causing necrosis and hepatocyte damage in the liver and kidney. Fibrosis lead to ectopic fat accumulation, resulting in non-alcoholic fatty liver disease (Byrne, 2013; Kwon et al., 2021b; Pafili and Roden, 2021). These histological changes were also observed in our study. Fibrosis due to exposure to flutriafol affected the morphological characteristics in liver, kidney, muscle, fat, and ileum tissue of pigs.
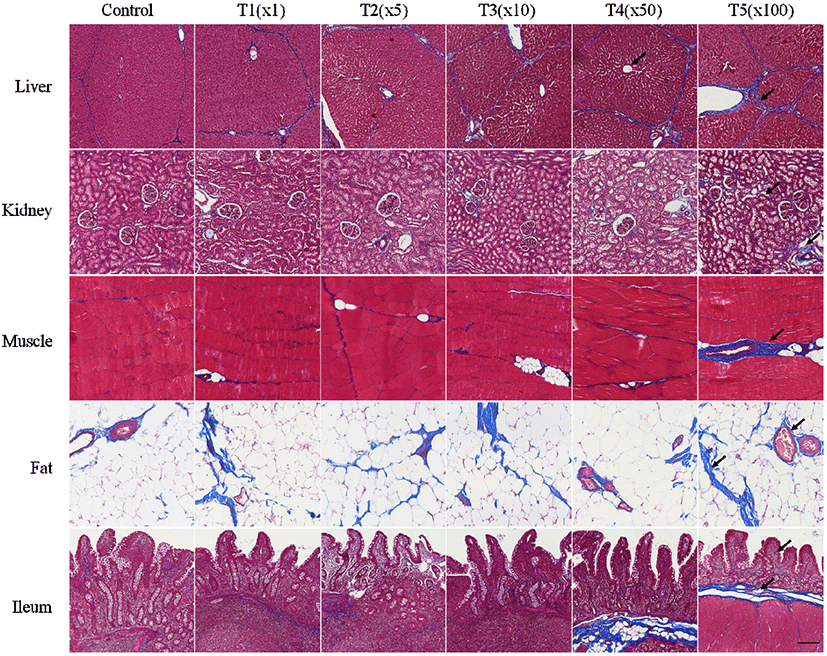
Conclusion
The results of the present study suggest that flutriafol exposure affects pig tissues (e.g., muscle, fat, blood, liver, kidney, and ileum), causing significant alterations in some biochemical parameters including BUN, CREA, ALT, and LIPA. In particular, the linear and quadratic equations for liver and blood showed a significant increase (p<0.05) after exposure to different flutriafol concentrations. Flutriafol also can lead to morphological changes related to fibrosis in several tissues. Therefore, these results indicate that pesticides such as flutriafol can provide the basis for risk assessment and safety based on maximum residue limits in pig meat and related products.