Introduction
A pesticide is a single substance or mixture of substances used to prevent and remove pests and pathogenic fungi causing diseases. Although pesticides have many beneficial effects, they can also produce fatal toxicity in plants and animals (Brittain and Potts, 2011; Igbedioh, 1991). Pesticides are classified by the World Health Organization (WHO), and they can have varying levels of toxicity to humans and animals following repeated or prolonged exposure (http://www.inchem.org). Exposure to pesticide may be indicated by skin, digestive, respiratory, and conjunctivital tissues. Pesticides found in food and feedstuff are an important influencer of the health of human and animals (Alengebawy et al., 2021; Mamane et al., 2015). Pesticides, including fungicides, herbicides, and insecticides, are sprayed directly onto plants to prevent unwanted bacteria and viruses; however, it is important to note that plants can uptake and metabolize these pesticides (Karabelas et al., 2009).
Fungicides are used as agents to protect humans and animals from various diseases caused by fungi. Metconazole, propiconazole, prothioconazole, and tebuconazole are some of the most commonly used and effective triazoles for field application (Klink et al., 2021). The triazole classes have been widely studied and shown to exhibit various toxic neurobehavioral effects in rats and mice (Allen et al., 2006; Moser and Macphail, 1989). Propiconazole, a triazole fungicide, is used in agricultural products and forage crops. The indiscriminate use of fungicides can trigger the evolution of fungicide-resistance (van den Bosch et al., 2014).
Fibrosis is caused by a normal wound healing, but organ dysfunction is affected by dysregulation fibrosis. Prolonged exposure to pesticides can cause liver damage (e.g., fibrosis or cancer) (VoPham et al., 2017). Fibrosis is characterized by excessive and abnormal deposition of extracellular matrix (ECM) components (Herrera et al., 2019; Uitto and Kouba, 2000). ECM may affect various organs, including liver, kidney, lung, and skin. Chronic activation of fibroblasts can result in large-scale deposition of fibrous ECM and excessive fibroblast/myofibroblast proliferation (Kendall and Feghali-Bostwick, 2014; Verrecchia and Mauviel, 2007).
The widespread use of azole fungicides can cause azole resistance, however, little is known about the effects of propiconazole exposure on pigs. Therefore, this study was conducted to investigate the potential effects of propiconazole residues in pig meat and meat products.
Materials and Methods
All animal experimental procedures were approved and reviewed by the Institutional Animal Care and Use Committee of the National Institute of Animal Science in Korea (No. 2019-1576).
Pigs (approximately 110 d; 70±2.4 kg) were purchased from the Darby breeding company (Anseong, Korea). A total of 18 castrated male pigs (Landrace×Yorkshire) were housed and separated in individual areas (2.1 m×1.4 m). The animals were fed according to the Korean feeding standard for pigs (2017), under a light-dark cycle (12:12 h) at constant temperature (21±2°C) and relative humidity (55±10%), with water provided ad libitum. Pigs were acclimated to these conditions for 14 d, after which, they were divided into six groups as follows: control, T1 (x1; 0.09 mg/kg bw/d), T2 (x5; 0.44 mg/kg bw/d), T3 (x10; 0.88 mg/kg bw/d), T4 (x50; 4.41 mg/kg bw/d), and T5 (x100; 8.82 mg/kg bw/d) (n=3). Propiconazole was properly mixed into the feed according to the concentrations determined based on body weights. Animals were identified by exposure to propiconazole twice daily for 28 d. At the end of the 28-d experimental period, all pigs were anesthetized with T61. After exsanguination, the tissue samples were quickly removed from blood, liver, kidney, ileum, muscle, and fat. For the histological study, a part of the tissues sample was fixed in 10% neutral buffered formalin (Sigma-Aldrich, St. Louis, MO, USA), while another part was flash frozen in liquid nitrogen and stored at −80°C for real-time PCR and residue analysis. The growth performance of pigs is listed in Table 1.
The liver, kidney, and ileum pieces fixed in 10% neutral buffered formalin (Sigma-Aldrich) were dehydrated in an increasing ethanol series (from 70% to 100%), embedded in paraffin, cut (5-μm thick) using a microtome, mounted on glass slides, and heated at 40°C for 1 h to attach to sections. These sections were then dewaxed in xylene, rehydrated with a decreasing ethanol series (from 100% to 70%) for 2 min, and washed with distilled water. Subsequently, tissue slices were stained in Masson’s trichrome (MT) staining reagents, according to the manufacturer’s protocol. All tissues were assessed by measuring fibrosis under the microscope at 100× magnification.
Total RNA from tissue samples (i.e., liver, kidney, muscle, and ileum) was extracted using the TRIzol reagent (Molecular Research Center, Invitrogen, Carlsbad, CA, USA), according to the manufacturer’s instructions. Briefly, total RNA was quantified using infinite M NANO (Tecan, Männedorf, Swiss) at 260 nm and checked on 1.0% (w/v) agarose gel, and cDNA synthesis was performed on samples diluted to 1 μg using a GoScriptTM reverse transcription kit (Promega, Madison, WI, USA), according to the manufacturer’s instructions. Real-time PCR was performed using QuantiTect SYBR Green PCR Master Mix (Qiagen, Hilden, Germany) with a CFX96 PCR system (Bio-Rad, Hercules, CA, USA), as previously described (Jeong et al., 2012). The target gene expression levels were normalized to that of glyceraldehyde 3-phosphate (GAPDH) and skeletal muscle alpha-actin (ACTA1) endogenous genes. Relative mRNA quantification was performed using the comparative 2(-Delta Delta CT) method according to the universal method. The primers were designed by integrating DNA technology (https://www.idtdna.com/), based on the nucleotide sequences published in GenBank and listed in Table 1.
The blood samples were collected without an anticoagulant tube and centrifuged at 700 g for 15 min. The serum was stored at −80°C until use. Glucose (GLU), creatinine (CREA), blood urea nitrogen (BUN), phosphate (PHOS), calcium (CA), total protein (TP), albumin globulin (ALB), alanine aminotransferase (ALT), alkaline phosphatase (ALKP), gamma glutamyl transpeptidase (GGT), total bilirubin (TBIL), cholesterol (CHOL), amylase (AMYL), and lipase (LIPA) content were measured with a VetTest chemistry analyzer (IDEXX, USA) with chem 17 clip, according to the manufacturer’s method.
To quantify propiconazole in pig tissues, 10 mL of distilled water was added to 2.0 g of the sample (2.0 mL of blood) collected at each site. Samples were allowed to stand for 10 min, before adding 20 mL of acetonitrile and 5 g of NaCl, stirring for 10 s using a vortex mixer, and shaking horizontally for 60 min. The supernatant was then centrifuged at 3,500×g for 5 min, and acetonitrile, an organic solvent layer, was used to resuspend the analysis sample. The samples were purified using primary-secondary amine (PSA) and C18. After adding about 50 mg of PSA and C18 into a microtube, 1 mL of the analysis sample was transferred to a microtube, mixed for about 1 min, and centrifuged at 12,000×g. The sample was filtered, 5 μL was injected into the liquid chromatography-tandem mass spectrometry (LC-MS/MS) apparatus, and the peak area on the chromatogram displayed was compared with that in a standard calibration curve to calculate the residual amount. A stock standard solution (1 mg/L) was prepared with propiconazole analytical standard (99.0% purity, 10 mg/L). Calibration solutions were prepared in seven different concentrations—0.001, 0.002, 0.005, 0.01, 0.02, 0.05, and 0.1 mg/L—with methanol. Five microliters of the five replicates of untreated samples (plasma, liver, kidney, muscle, fat) were injected into the LC-MS/MS, and the peak area on the chromatogram was monitored. The minimum detectable amount of propiconazole in LC-MS/MS was 0.005 ng, and the quantitative limit of the assay was 0.01 mg/kg. Chromatographic analyses were carried out using an Exion LC system consisting of a QTRAP 4500 mass spectrometer (SCIEX, Framingham, MA, USA). Briefly, the conditions were as follows: column (100×2.0 mm, 3.0 μm) maintained at 40°C, mobile phase composition (10 mM ammonium acetate and methanol), linear gradient mode (from 20% to 90% methanol), flow rate (0.1 mL/min), and product ion analysis (collision energy 40 eV).
The statistical analyses of growth performance, biochemical analysis, and expression levels were performed by one-way ANOVA (Prism 5.01, San Diego, CA, USA), followed by Tukey’s multiple comparison post-hoc test. Differences between control and treatment groups were considered significant if p<0.05. Results are presented as mean and SEM.
Results and Discussion
The effects of treating pigs with propiconazole for 28 d on the growth performance of finishing pigs are summarized in Table 2. A previous studies showed that propiconazole did not effect on body weight in Sprague–Dawley rats (Costa et al., 2015; Kwon et al., 2021) and mice (Sun et al., 2005). Therefore, the body weight (initial and final), average daily weight gain, average daily feed intake, and feed conversion ratio were not affected by propiconazole exposure.
Blood biochemical tests showed differences in propiconazole concentrations among control and treatment groups (Table 3). GLU, CREA, BUN, PHOS, TP, ALB, globulin (GLOB), ALT, ALKP, TBIL, CHOL, and LIPA were differentially regulated (p<0.05) among the groups, while CA, GGT, and AMYL were unaffected. TP was significantly increased in all the groups compared with normal range, except the T1 group. ALB and TBIL were higher than the normal range in all groups (p<0.05). ALT showed a greater increase in the treatment group compared with the control, except in the T2 and T3 groups. However, ALKP was significantly decreased in the exposure groups compared with the control, except for in the T3 group. There were no significant differences in the other biochemical parameters (i.e., CA, GGT, and AMYL). A 2D analysis of the principal component analysis (PCA) score plot did not show any differences between the control and treatment groups (Fig. 1A). However, PCA score plots showed a separation on the three-dimensional analysis in all groups (Fig. 1B).
Normal range value: GLU (85–160), CREA (0.5–2.1), BUN (6–30), PHOS (3.6–9.2), CA (6.5–11.4), TP (6.0–8.0), ALB (1.8–3.3), GLOB (2.5–4.5), ALT (9–43), ALKP (92–294), GGT (16–30), TBIL (0.1–0.3), CHOL (18–79), AMYL (271–1198), LIPA (10–44).
GLU, glucose; CREA, creatinine; BUN, blood urea nitrogen; PHOS, phosphate; CA, calcium; TP, total protein (TP=ALB+GLOB); ALB, albumin globulin; GLOB, globulin; ALT, alanine aminotransferase; ALKP, alkaline phosphatase; GGT, gamma glutamyl transpeptidase; TBIL, total bilirubin, CHOL, cholesterol; AMYL, amylase; LIPA, lipase. All traits in the table were analyzed by one-way ANOVA with Tukey’s multiple comparison test.
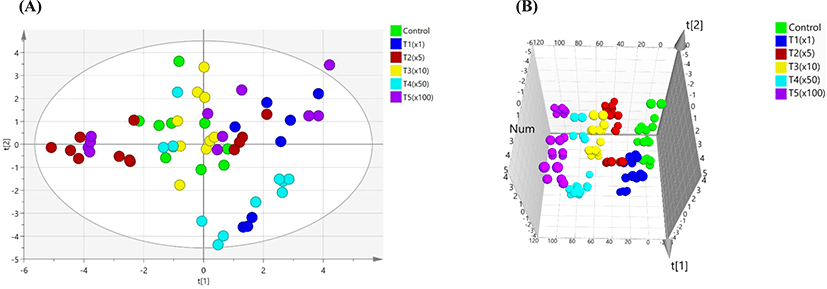
PCA was used to distinguish between control and treatment groups. In this study, we analyzed to searching the indicators on blood biochemical test by PCA due to individual results are not possible to find important indications. Therefore, variable importance in the projects (VIPs) plot analysis was performed based on PCA model. PCA analysis was able to differentiating between of Pale Soft Exudative (PSE) meat and normal by measuring their blood biochemical parameters. Among these parameters, creatine kinase (CK), lactate dehydrogenase (LDH), aspertate aminotransferase (AST), GLU, and lactate (LAC) were associated with PSE meat as good indicators (Qu et al., 2017). Blood biochemical tests can reveal important information in laboratory feeding experiments in human and animals. Stress can induce differences in biochemical markers in the transamination pathway and can contribute to toxic stress induced by pesticide (Séralini et al., 2007; Velisek et al., 2011). Herein, for variable ranking in biochemical values, VIP with the PLS-DA model extracted a value higher than 1. The VIP score was as follows: BUN (1.36), CHOL (1.28), PHOS (1.18), ALKP (1.16), and ALT (1.16). The use of pesticides also affects the immune system (Huang et al., 2020; Yang et al., 2020). The liver is onsidered an important organ in the body’s defense system against toxins, while the kidney is involved in the excretion of xenobiotics and metabolites synthesized as a result of damage induced by toxic xenobiotics, such as pesticides (George et al., 2017; Lushchak et al., 2018). Serum cholesterol is influenced by dietary intake and production in the liver from fatty acids. ALKP refers to a group of enzymes that catalyze the hydrolysis of phosphate groups (Sharma et al., 2014). ALT is a cytosolic enzyme that is almost exclusively found in hepatocytes (Kim et al., 2008). Urea is synthesized by ammonia in the liver and is subsequently excreted through the kidneys (Wang et al., 2014). The activities of aspartate AST, ALT, GGT, ALKP, and LDH were all significantly higher in the exposed group (Cu-based pesticide) (Arnal et al., 2011). The nominal concentrations of propiconazole in rainbow trout used were E1 group according to environmental concentration, E2 group, and E3 group. Long-term exposure to propiconazole resulted in increases of levels of NH3 and GLU, and activities of CK, LDH, ALT, and AST (Li et al., 2011). Blood biochemical profiles provide important information for the internal environment in in vivo (Fırat et al., 2011). In this study, BUN was one of the potential biochemical markers, which considerably elevated with exposure to propiconazole compared with the non-exposure group.
Fig. 2 shows the recoveries of the pesticides, ranging between 77% and 109.2%, depending on the propiconazole spiking concentrations (0.01, 0.1, and 0.5 mg/kg). The recovery rates in the blood (Fig. 2A), liver (Fig. 2B), kidney (Fig. 2C), muscle (Fig. 2D), and fat (Fig. 2E) tissues were 70–90, 93–102, 79–89, 75–80, and 80–92, respectively. The distribution of apparent recoveries in terms of the individual value in five tissues (Fig. 2A–E) and the average value (Fig. 2F) of each tissue, including blood, liver, kidney, muscle, and fat, are showed under repeatability conditions. Polynomial regression was evaluated under different limits of propiconazole (Fig. 2).
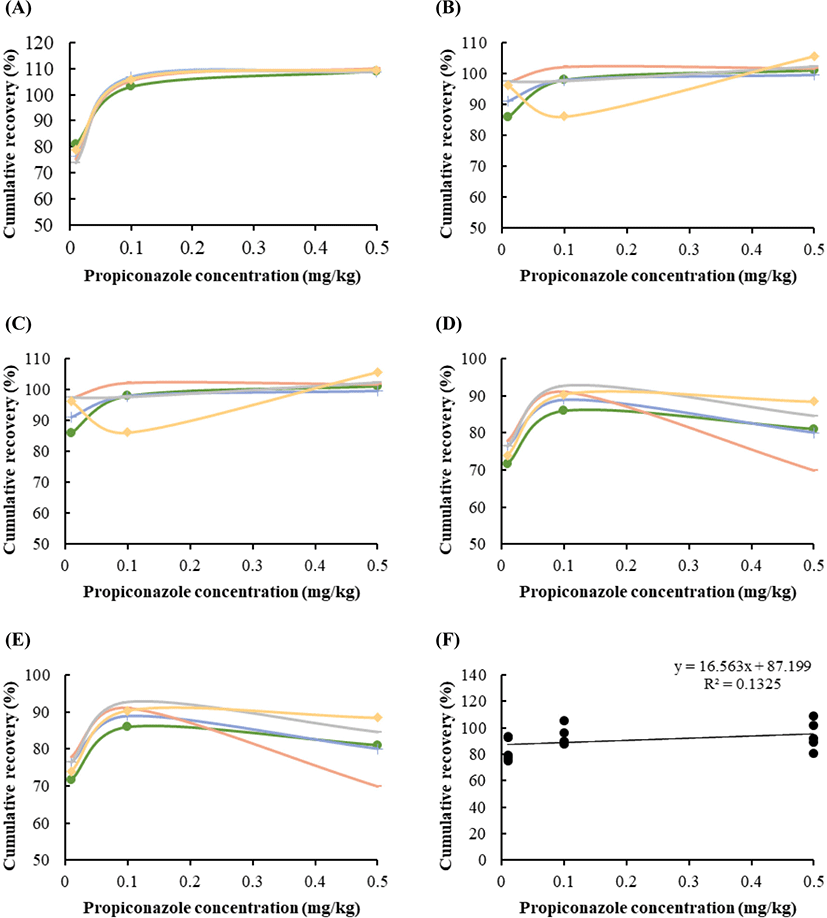
Linear and quadratic equations modeling propiconazole exposure were developed to estimate the maximum residue in the liver (Fig. 3A), kidney (Fig. 3B), and fat (Fig. 3C). The levels in blood and muscle did not significantly differ in any experimental group because the concentration was below the detection limit. However, the residual levels in the liver, kidney, and fat tissue were measured by investigating the propiconazole concentration gradient. The quadratic equations based on the propiconazole concentration in liver showed high r2 (0.9996) and significant differences (p=0.021; Figs. 3A). The equations for muscle (r2=0.9589) and fat (r2=0.9934) tissue did not differ based on the propiconazole concentrations (Figs. 3B and 3C).
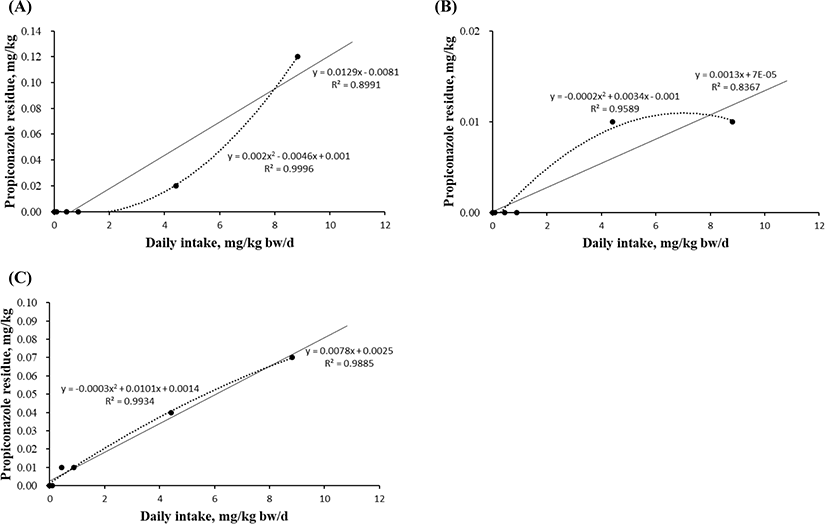
Recovery is a parameter that combines the analyte from the sample matrix and matrix effect, and is also referred to as the “process efficiency”. In general, the apparent recovery should be 70%–120% (Sharma et al., 2012; Steiner et al., 2020). The quantitative recoveries were measured in pork liver (71%–80%) and kidney (82%–84%); these values were, therefore, suitable. Taken together, our results suggest that exposure to propiconazole results in accumulation in meat production, except the bloos and muscle.
To compare the mRNA expression of fibrosis-related genes, we performed RT-PCR for these genes in the liver, kidney, muscle, and ileum tissues of pigs according to their exposure to propiconazole. Herein, the expression level of eight genes was measured between the control and treatment groups (Fig. 4). The gene expression of transforming growth factor beta 1 (TGFb1), collagen type I alpha 1 chain (COL1A1), and collagen type III alpha 1 chain (COL3A1) increased significantly in liver tissue up to 100 fold following propiconazole exposure (Fig. 4A). Similarly, the expression of TGFb1 and COL1A1 increased significantly in kidney tissue up to 100 fold following propiconazole exposure (Fig. 4B). The gene expression of TGFb1, COL1A1, and COL3A1 increased significantly in fat tissue up to 100 fold in the T5 group, while that of periostin (POSTN) also increased 50 fold from the T4 to T5 groups following propiconazole exposure (Fig. 4C). The gene expression of TGFb1, connective tissue growth factor (CTGF), COL1A1, COL3A1, and bone morphogenetic protein 7 (BMP7) increased significantly with a concentration-dependent response exposed to propiconazole in ileum tissue (Fig. 4D).
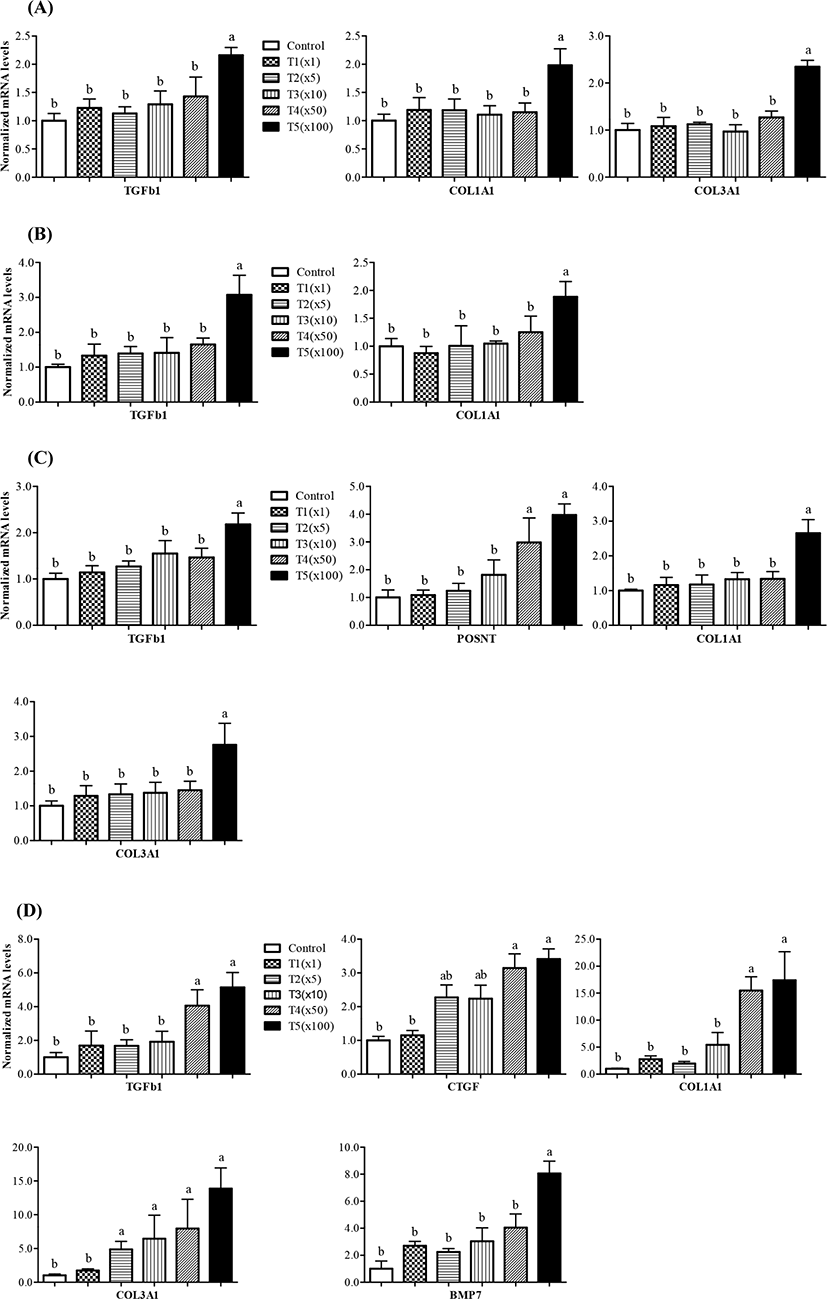
Thus, propiconazole affects the expression of fibrosis-related genes, while not affecting Activin A receptor type 2A (Acta2a) and TIMP metallopeptidase inhibitor 1 (TIMP1) gene expression (data not shown). The exposed to propiconazole to human liver cells and rat liver can lead to liver fibrosis (Kwon et al., 2021). Activation of TGFb1/Smad signaling is known as inducer of kidney fibrosis (Meng et al., 2015). BMP7, which is receiving attention as a fibrotic disease agent, is critically involved in renal morphogenesis and development through TGFb signaling mediated SMAD (Godin et al., 1998; Manson et al., 2015).
Anti-CTGF treatment can be applied to various types of fibroproliferative disorders (Wang et al., 2011). The mechanism of their interaction remains unclear, although TGFb acts as a mediator of CTGF. Therefore, CTGF is a marker of disease states and fibrosis (Bennewith et al., 2009; Chen et al., 2001; Ivkovic et al., 2003). COL1A1, COL3A1, and TIMP1 genes was shown to indicate fibrosis with a significant increase in S. japonicum infection (Tao et al., 2018). Periostin is a potential biomarker for disease such as lung fibrosis (Naik et al., 2012). Periostin also affects cellular function by binding to matrix proteins and cell receptors (Horiuchi et al., 1999). TGFb mediated periostin is produced by structural and inflammatory cells and is upregulated during fibrotic responses in mice and humans (Naik et al., 2012). Propiconazole-induced cell proliferation is involved in cytotoxicity and regenerative proliferation (Hester et al., 2006; Hester et al., 2012). Overall, the results of the present study indicate that propiconazole residues affected the variable expression of genes involved in fibrosis in different tissues regardless of pig weight.
We further investigated histological changes following exposure to propiconazole in pig tissues (e.g., liver, kidney, and ileum) using MT staining (Fig. 5). Propiconazole exposure was shown to increase collagen fiber destruction and deteriorate fibrosis. The portal areas containing the portal vein, hepatic artery, and bile duct of the liver were stained blue by MTs staining. Moreover, the fiber depositions of the lobular boundary also showed prominent staining. The expansion of the portal canals and lobule boundary also showed propiconazole accumulation. The Bowman’s space of glomerulus and glomerulosclerosis in kidney tissues was stained blue according to concentration. The villus and lamina propria of the ileum had deteriorated and exhibited prominent blue staining for propiconazole compared with that of the control.
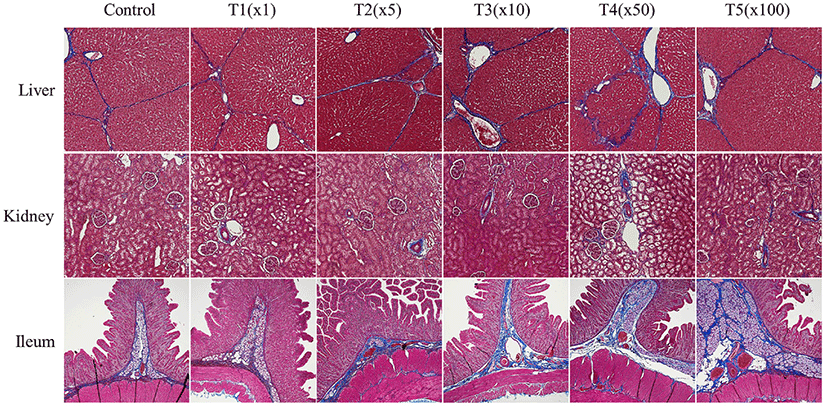
Following exposure to pesticides, the liver and kidneys have been shown to exhibit a change in the vacuoles in the proximal tubules, causing necrosis and hepatocellular damage, respectively (Eid, 2017; Lenz et al., 2018). Multi-enzyme may benefit more than single or two enzyme supplement to improving gut health (Ramani et al., 2021). These changes can be observed within our study. Fibrotic changes were affected by exposure to propiconazole in liver, kidney, and ileum of finishing pigs. Taken together, our results suggest that exposure to propiconazole causes severe morphological changes.
Conclusion
Propiconazole can be used as an anti-viral and antifungal agent to protect feed products from fungal invasion. In this study, we found that liver, kidney, and fat tissues contained residual levels of propiconazole, whereas the blood, muscle, and ileum tissues had lower levels. Propiconazole exposure also induced morphological and gene expression changes related to fibrosis. This study provides important information regarding the maximum residue limits of propiconazole, and its safety in meat products.