Introduction
Meat is a good source of protein, including essential amino acids, and contains B vitamins as well as minerals such as iron, potassium, and magnesium (Warriss, 2000). Accordingly, high-quality meat products have attracted consumers’ interest due to their high nutritional values and palatability. Sausages are considered an ideal food because they are a convenient, ready-to-eat food that can be prepared using the edible parts of the produced carcass and have the nutritional benefits of raw meat (Puolanne, 2010).
Salt and fat play important roles, such as improving sensory attributes and functional properties in processed meats (Weiss et al., 2010). Salt is an essential additive because of its effect on texture, cooking yield (CY), water-holding capacity (WHC), sensory quality, and shelf-life of meat products (Honikel, 2010; Ruusunen et al., 2005). Typical cooked sausages (frankfurters and wieners) contain 15%–30% fat (Puolanne, 2010), which improves the texture, juiciness, and palatability of processed meats (Tobin et al., 2013).
Although salt and fat perform key functions in meat products, their excessive intakes are dietary risk factors for various diseases. Hypertension can be caused by high salt intake, and it is known to be a risk factor for cardiovascular diseases and stomach cancer (Aburto et al., 2013; Pearson and Wolzak, 1982). Fat is a high-energy source and a necessary nutrient for the human body. Although fat is a good nutritional source for humans, fat-rich diets are directly related to the risk of obesity, and it can lead to colon cancer and cardiovascular diseases (Jiménez-Colmenero et al., 2001). Based on these facts, the World Health Organization (WHO) suggested that the daily intake of salt should be less than 5 g (WHO, 2003). As for fat, there have been recommendations to reduce the intake of saturated fatty acids to less than 10% of total energy intake and trans fatty acids to less than 1% of total energy intake (WHO, 2023). Nowadays, as consumers’ interest in health gradually increases, the trend is reducing the added levels of salt, fat, and cholesterol in processed meats (Yang et al., 2007). Thus, although salt and fat have important functional properties in the manufacturing of meat products, healthier meat products with a reduction of fat and salt should be developed for the health of consumers.
Faba bean (broad bean, Vicia faba L.) is grown as a staple food in North African and Middle Eastern countries. It is a pulse crop that can be cultivated without an irrigation system and is resistant to cold weather and heavy rainfall (Multari et al., 2015). Faba bean is a useful and sustainable protein source with nutritional and functional properties, and faba bean flour, concentrate, and isolate are known to have high potential in the food industry (Sharan et al., 2021). Several studies have shown that some functionalities of faba bean protein, such as WHC, are comparable to other legume proteins, such as soy, pea, and lentil, which are widely used as an extender and binder in industrial food application. For instance, Marti-Quijal et al. (2018) reported that the addition of faba bean protein into cooked turkey breasts improved hardness values compared to those with lentils. Additionally, Johansson et al. (2022) reported that because of its useful functional properties, faba bean has the potential to be an alternative to soybean in food applications.
Microbial transglutaminase (MTG) is an enzyme that catalyzes the formation of an isopeptide bond, an ε-(γ-glutamyl)lysine cross-link, between a glutamine (Gln) residue in a peptide chain and ε-amino group of a lysine (Lys) residue in a peptide (Mycek et al., 1959). Because MTG catalyzed reactions can modify the functional properties of protein foods, the enzyme has been used to improve functional properties, such as WHC, elasticity, and solubility of textured products (Zhu et al., 1995). Yong et al. (2020) reported that the addition of both soy protein isolate (SPI) and MTG could increase the textural properties of reduced-salt meat emulsions by contributing to gelation via cross-linking. According to Lee and Chin (2013a), the incorporation of mung bean flour to low-salt pork model sausages might interact with MTG, and it could enhance water retention and textural properties. Because faba bean contains abundant Gln and Lys for cross-linking (Azaza et al., 2009; Eckert et al., 2019), the combination of faba bean protein isolate (FBPI) and MTG may be suitable for the improvement of functional properties of processed meat products with reduction of fat and salt. Few previous studies applied for meat products such as low-fat bologna (Wei, 2019) and minced meat (Ramos-Diaz et al., 2022) with faba bean protein alone, but they didn’t apply it for the MPG and the combination of FBPI and MTG into reduced-salt pork sausage. Therefore, the aim of this study was to evaluate the effect of FBPI and MTG on the structural changes of pork myofibrillar protein gel (MPG) and quality characteristics of reduced-salt, low-fat pork model sausage (LFMSs) for the manufacture of healthier meat products.
Materials and Methods
Pork loin (Landrace×Yorkshire×Duroc three-way cross-breed pig) was purchased from a retail market (Hyundai Distribution, Gwangju, Korea). To extract the myofibrillar (salt-soluble) proteins (MPs), the raw meat was cut into cubes (1–2 cm3) after removing the external fat and connective tissues. The trimmed meats were vacuum-packed and stored frozen at –20°C until extraction of MPs. The FBPI was supplied by Yantai Shuangta Food (Zhaoyan, China). The MTG (ACTIVA TG-TI) was purchased from Ajinomoto (Tokyo, Japan).
The frozen pork loin cubes were thawed for about 12 h in a refrigerator at 4°C. Thawed pork loins were blended with buffer solution [0.1 M sodium chloride (NaCl) and 50 mM sodium phosphate; pH 6.25]. The blended mixture was centrifuged at 1,660×g (Supra 22, Hanil Scientific, Kimpo, Korea). After the supernatant of centrifuged samples was removed, the collected precipitate and buffer solution were mixed and centrifuged again (at least three times). The mixture of precipitate and 0.1 M NaCl buffer solution (pH 6.25) was filtered and centrifuged to remove connective tissue. The protein and salt concentration of MP was analyzed and adjusted to 4% protein at 0.45 M salt with NaCl/phosphate buffer solution. Table 1 shows the formulation of pork MPGs with FBPI or MTG.
Ingredients (%) | CTL | MTG | FBPI | FBTG |
---|---|---|---|---|
Myofibrillar protein mixture | 80.0 | 80.0 | 80.0 | 80.0 |
Buffer solution | 20.0 | 19.0 | 18.0 | 19.0 |
FBPI | - | - | 1.00 | 1.00 |
MTG | - | 1.00 | - | 1.00 |
Total | 100.0 | 100.0 | 100.0 | 100.0 |
The CYs (%) were measured by heating the sample in a water bath (WB-22, Daihan Scientific, Seoul, Korea) from 20°C to 80°C. After heating, samples were stored at 4°C for 12 h for cooling and kept at room temperature for 30 min. The exudate on the surface of MPG was removed, and the weight was measured. CY was calculated by the following formula:
The cooked MPGs were used for measurement of gel strength (GS; gf) after determination of CY using an Instron Universal Testing Machine (Model 3344, Instron®, Norwood, MA, USA). Data were recorded by the Merlin program (Instron®). The breaking force (gf) were measured at the first peak values by a compression test with a 500 N load cell of 9 mm diameter (50 mm/min cross-speed).
The raw samples intended for measurement of the viscosity of MPG were placed aside from the other samples before heating. The viscosity was expressed by recording the changes in shear stress (Pa) with the increase of shear rate (1/s) in the range of 0 to 600/s with a cylinder-type rotational rheometer (RC30, Rheotec Messtechnik, Dresden, Germany). The results of viscosity were plotted as a graph using Excel (ver. 2016; Microsoft, Redmond, WA, USA).
The protein surface hydrophobicity of MPG was measured using the method of Chelh et al. (2006). The samples were adjusted to 10 mg/mL of protein concentration with MPGs and 20 mM sodium phosphate buffer (pH 6.00). The MPG solution (1 mL) and bromophenol blue solution (200 μL, 1 mg/mL in distilled water) were mixed, and the blank was prepared by replacing MPG with 1 mL of 20 mM sodium phosphate buffer. The samples and blank were agitated for 10 min with a vortexer (KMC-1300V, Vision Scientific, Daejeon, Korea) were then centrifuged (VS-5500, Vision Scientific) at 2,000×g for 15 min. The OD values of samples and blank were measured at a wavelength of 595 nm using a spectrophotometer (X-ma 1200, Human Corporation, Seoul, Korea). The hydrophobicity values were derived by substituting the OD values into the following formula:
The sulfhydryl groups were determined using the modified Ellman’s method (Beveridge et al., 1974). The unheated sample (0.1 g) was blended with distilled water (8.4 mL), 4 mg/mL of 5,5’-dithiobis-2-nitrobenzoic acid (DTNB) solution (0.5 mL), and Tris-Gly 8 M urea (1 mL). The blank was prepared by replacing the sample with 20 mM phosphate buffer (pH 6.25). Then, the mixtures were incubated for 5 min at room temperature before measurement of their OD values at a wavelength of 412 nm with a spectrophotometer. The sulfhydryl group values were derived using a molar extinction coefficient of 13,600 M–1cm–1.
Sodium dodecyl sulfate-polyacrylamide gel electrophoresis (SDS-PAGE) was performed to investigate the effect of added FBPI or MTG on the polymerization of MPG. The sample was adjusted to a 1% protein concentration, combined with sample buffer (Model 161-0737, Bio Rad Laboratories, Hercules, CA, USA), and loaded on a 10% acrylamide separating gel (0.375 M Tris, pH 8.80) and 4% acrylamide stacking gel (0.125 M Tris, pH 6.80; Laemmli, 1970). Electrophoresis was carried out at 150 V for 1.5 h to separate protein bands. A standard marker (Model 161-0318) was used for determining the relative molecular weight (MW) of the proteins in the samples.
The microstructure of MPG was visualized with a low-vacuum scanning electron microscope (JSM-6610LV, JEOL, Tokyo, Japan) in order to compare the three-dimensional (3D) structure among treatments. Samples were cut into a cuboidal shape (3 mm3) and fixed by soaking for 24 h in 2.5% glutaraldehyde in 0.1 M sodium phosphate buffer (pH 7.00) at 4°C. Post-fixed samples were immersed in osmium tetraoxide (OsO4) in 0.1 M sodium phosphate buffer (pH 7.00) for 5 h. After that, samples were dehydrated using various concentrations (50%, 60%, 70%, 80%, 90%, 100%) of ethanol solution and acetone solution for 10 min per solution. Dehydrated samples were gold-coated using an auto-sputter coater (Cressington Scientific Instruments, Watford, UK). The microstructure of each sample was observed at a fixed magnification ×1,000.
Pork ham (Landrace×Yorkshire×Duroc three-way cross-breed pig) was purchased from a local retail market in order to manufacture LFMSs. Raw ham muscle was trimmed to remove connective tissue and external fat, then stored in a freezer at –20°C until used for the preparation of LFMS. FBPI was hydrated with water (1:4) and used as a binder in the manufacture of reduced-salt pork sausages. The MTG consisted of 1% enzyme, 5% sodium polyphosphate, 5% sodium pyrophosphate, 0.5% sodium ascorbate, and 88.5% lactose (ACTIVA TG-S, Ajinomoto).
The formulation of manufactured low-fat pork sausages is shown in Table 2. Ground pork ham and fat replacer were comminuted with ice water for 30 s. Then, the mixture was homogenized with salt, sodium tripolyphosphate, and erythorbate for 1 min. Next, meat batter and ice water were mixed again. After that, the batter was stuffed into conical tubes (SPL Life Sciences, Pocheon, Korea) and cooked in a water bath (WB-22, Daihan Scientific) until the internal temperature reached 72°C. Finally, cooked pork sausages were cooled in an ice box and stored in a refrigerator at 4°C until analyzed.
The pH values were measured using a pH meter (Model 340, Mettler-Toledo, Greifensee, Switzerland). Five recordings were taken per sample, and the mean value was calculated. Before measurements the instrument was calibrated against pH 4.01 and 7.00 buffers.
The color values were determined using a CIE color reader (Model CR-10, Minolta, Osaka, Japan). CIE L*, CIE a*, and CIE b* were measured six times, and a mean value was calculated. Before measurement, the instrument was calibrated against a standard white color plate (CIE L*=94.8, CIE a*=1.0, and CIE b*=0.1).
The cooking loss (CL; %) was measured by deriving the weight of samples before and after cooking to investigate WHC and processing yield according to the following formula:
The sausage sample was prepared by cutting it into a cube shape of 1.5 g for the measuring expressible moisture (EM; %). Each sample was wrapped with three Whatman #3 filter papers (GE Healthcare, Little Chalfont, UK), placed into a conical tube, and centrifuged at 1,660×g (VS-5500, Vision Scientific) for 15 min. After that, the weights of the sample and filter paper were measured, and EM was calculated using the following formula:
The sausage sample with a height of 1.3 cm and a diameter of 1.25 cm was prepared for texture profile analysis. The hardness (gf), springiness (mm), gumminess, chewiness, and cohesiveness values of 10 samples were determined with an Instron Universal Machine (Model 3344, Instron®). A two-bite test was performed at a speed of 300 mm/min with a load cell of 500 N using a bellow compression probe.
The moisture, protein, and lipid contents of LFMSs were measured according to the Association of Official Analytical Chemists (AOAC, 2000). The total moisture content (%) was determined by the dry oven method. Each homogenized sample was placed in a filter paper thimble and oven-dried at 100°C for 16–18 h. The crude fat content (%) was measured by the Soxhlet extraction method using the sample used for moisture analysis. The sample with petroleum ether was heated in a Soxhlet extractor at 60°C–80°C for 5 h and oven-dried again. After that, the fat content was derived by calculating the weight loss after extraction and drying. The Kjeldahl method was performed to determine crude protein content (%) by quantifying nitrogen in the sample.
All experiments for Study I and Study II were conducted in triplicate for each treatment. The data were expressed as means and SDs. One-way analysis of variance with Duncan’s multiple range test was performed to investigate the differences between treatments as a factor at a significance level of 95% using IBM SPSS Statistics 26 (IBM, Armonk, NY, USA).
Results and Discussion
The CYs (%) of MPGs with FBPI alone or in combination with MTG are shown in Table 3. The addition of FBPI increased the CY of MPG, whereas the addition of MTG decreased the CY (p<0.05). However, MPGs with both FBPI and MTG had similar CY values to those of CTL (p>0.05). Legume proteins incorporated into meat products as a binder during cooking have been shown to increase CY by modifying protein structure (Jang and Chin, 2011). Jang and Chin (2011) reported that MPGs with red bean protein isolate (1%) had higher CY than those without red bean protein isolate, and they concluded that red bean has an abundance of hydrophilic amino acids, such as glutamic acid (Glu) and aspartic acid (Meng and Ma, 2002). Like red bean, faba bean also contains and abundance of Glu and Asp, and its Glu content was reported to be the highest among the nonessential amino acids, although the content varied considerably by genotype (Multari et al., 2015). In addition, Fernández-Quintela et al. (1997) reported that legume proteins such as pea, faba bean, and soybean have the water absorption capacity interrelated to gel formation, and water and oil absorption capacities of FBPI were higher than those of SPI and pea protein isolate (PPI). Jang et al. (2015) reported that pork MPGs induced by MTG (0.1%, 0.5%, and 1.0%) had lower CY than those without MTG. These previous results were similar to the present study because the addition of MTG might lead to the release of water in MPGs by cross-link during accelerating protein-protein interaction (Ahhmed et al., 2009). Therefore, MTG might decrease the CY of MPGs, but the combination of FBPI and MTG into MPG could alleviate water release during cooking.
As shown in Table 3, the GS (gf) values of CTL were the lowest among all treatments (p<0.05). The GS values of MPGs with MTG or FBPI increased dramatically compared to CTL. However, the GS values of MPGs containing both FBPI and MTG were improved beyond those of other treatments (p<0.05), which indicated that FBPI contributed as a substrate for MTG in this study. Sun and Arntfield (2012) reported that the addition of PPI increased the GS values of a chicken MP. Plant-based proteins derived from legumes contain storage proteins (globulins), such as conglycinin (7S) and glycinin (11S), which show excellent physicochemical properties (Kimura et al., 2008). According to Hettiarachchy et al. (2013), the interaction of these proteins generates soluble aggregates, which could be a key factor in improving the gelation properties. Because faba bean also contains 7S and 11S globulins, FBPI has the potential to improve the rheological properties of the protein structure fraction (Multari et al., 2015). Pietrasik et al. (2007) reported that pork meat gels mediated by MTG had higher GS values, regardless of the added non-meat protein types, such as sodium caseinate, blood plasma, SPI, and gelatin. The addition of legume proteins, such as red bean and soybean, combined with MTG showed higher GS compared to the inclusion of protein or MTG alone (Jang et al., 2015). These reports suggest that the improved GS can be primarily attributed to MTG-mediated cross-linking between Gln and Lys of legume proteins, such as soy protein. Thus, the combination of FBPI and MTG could improve the GS of MPG by promoting gelation thorough protein-protein interactions, such as the cross-linking of globulins (Jiang and Xiong, 2013).
Table 3 shows the protein surface hydrophobicity of MPGs as affected by the addition of FBPI and MTG alone or in combination. Surface hydrophobicity was decreased by the incorporation of MTG, but it was increased by the addition of FBPI alone compared to CTL (p<0.05). The protein surface hydrophobicity is closely related to the WHC of protein structure; higher water retention tends to be associated with a higher surface hydrophobicity (Lee and Chin, 2020; Zayas, 1997). High hydrophobicity can contribute to improve WHC and GS by binding proteins and stabilizing the three-dimensional structure of gel (Lu et al., 2022). Chen et al. (2023) reported that the surface hydrophobicity and WHC of MP extracted from yellow croaker increased with increasing pea protein concentration. Storage proteins such as 7S (vicilin) and 11S (legumin) globulins contained in faba bean are connected by hydrophobic interactions and disulfide bond (Liu et al., 2022). These reports might support the results of present study in that CY and GS values of MPGs increased by the addition of FBPI. The addition of MTG can improve the WHC, but the results of the present study showed a decrease in the hydrophobicity values of MTG-meditated MPGs with added protein. It might be that the hydrophobic residues exposed at the protein surface were buried by the action of MTG, which would have altered the protein structure (Zhang et al., 2020). Nio et al. (1986) reported that the addition of MTG caused the gelation of protein by covalent bonds, not hydrophobic interactions and hydrogen bonds. The contrasting effects of FBPI and MTG on the hydrophobicity of MPGs indicated that both additives would affect the protein structure in this study.
As shown in Table 3, CTL had the highest sulfhydryl groups among all treatments (p<0.05). There were fewer sulfhydryl groups in MPG containing FBPI and MTG than those MPG with MTG alone (p<0.05). However, no difference was detected between the sulfhydryl groups of FBPI and FBTG (p>0.05). These results showed that the addition of FBPI or MTG alone might decrease the sulfhydryl groups of MPGs, and the combination of FBPI and MTG decreased the sulfhydryl group content compared MTG or FBPI alone. Because the exposure (decrease) of sulfhydryl groups and the increase of disulfide bonds are important factors for the formation of the unfolding structure of proteins, the sulfhydryl groups play an important role in developing the functional properties of MPs (Hamada et al., 1994; Zhang et al., 2015). Liu et al. (2000) concluded that the content of sulfhydryl groups of chicken MP with SPI was lower than those of the CTL, resulting in changes in the number of disulfide bonds due to 7S and 11S globulins, which are the main components of legume proteins. Lin et al. (2019) reported that the addition of SPI and peanut protein isolate increased the disulfide bonds of red bream MPs. The addition of MTG showed a decrease in total sulfhydryl groups of MP from silver carp by unfolding protein molecules (Li et al., 2020). Thus, the addition of FBPI and MTG could improve the functional properties of the proteins matrix by exposing sulfhydryl groups and facilitating disulfide bond with cross-linking in this study.
The shear stress values of MPGs as a function of shear rate are shown in Fig. 1. MPGs with MTG tended to have higher shear stress values than those of MPGs without MTG at the initial shear rate. The shear stress values of FBTG tended to be higher than those of MPG with MTG alone at about 250–700 1/s of shear rate, whereas the final shear stress values of MTG treatments were not different. MPG with FBPI had higher shear stress values than CTL at all shear rates. These results indicated that the addition of FBPI or MTG could increase the viscosity of MPGs, but this effect of FBPI might be lower than that of MTG. The addition of legume proteins can increase the viscosity of the protein network by entrapping water, and the viscoelastic abilities of legume protein products play a critical role in the gel formation of emulsions (Goldstein and Reifen, 2022). Because faba bean is a good source of globulins, which can form high viscosity by crystallization, it is able to strengthen the formation of protein structure (Mohanan et al., 2020; Suchkov et al., 1997). Hong and Chin (2010) reported that the addition of MTG increased the apparent viscosity of MPGs, regardless of the addition of sodium alginate or salt concentration. It might be due to the protein solubilization with formed covalent bonds between Gln and Lys (Moreno et al., 2008). The incorporation of FBPI and MTG in MPG might have the potential to improve the functional properties, including viscosity.
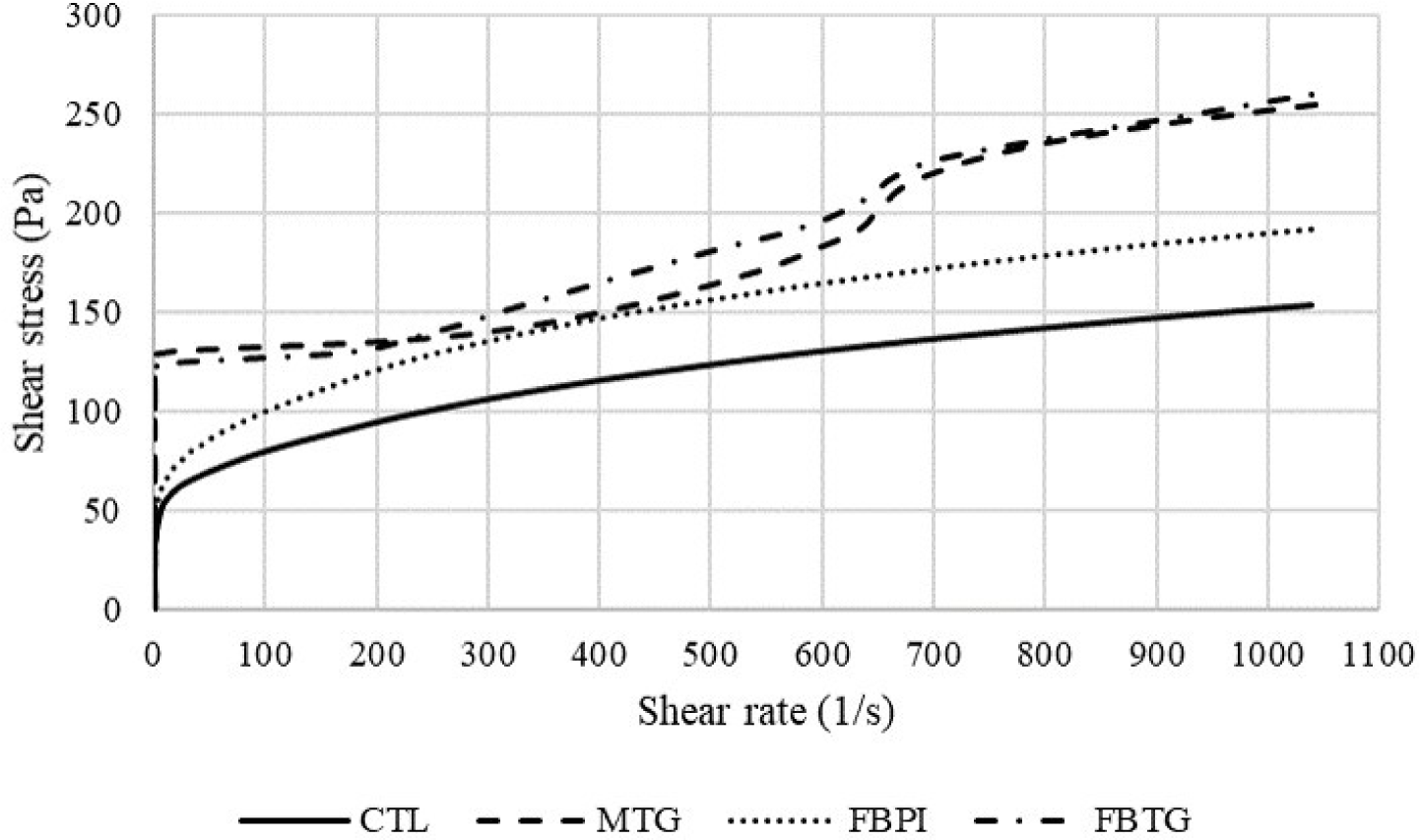
The protein patterns of MPGs with FBPI and MTG alone or in combination are presented in Fig. 2. MPGs with MTG had thinner myosin heavy chain (MHC) bands than those without MTG. Biopolymers formed from the interaction of MHC with MTG appeared at the top of the SDS-PAGE gel. Lee and Chin (2013b) reported that biopolymer bands appeared at high MW in profiles of MPGs treated with MTG and mung bean protein isolate. MPGs containing FBPI showed specific protein patterns between MWs of 43 and 50 kDa, but this was not observed in MPGs without FBPI. Bühler et al. (2020) reported that 7S and 11S globulins had MWs of 46–55 and 38–40 kDa in the protein pattern of faba bean protein concentrate, respectively. Several studies reported that combinations of muscle proteins with legumes, such as soy, pea, and kidney bean, showed detectable electrophoretic changes, such as the presence of globulin fractions, hydrophobic interactions, myosin aggregation, and intramolecular associations (Jiang and Xiong, 2013; Sun and Arntfield, 2012; Wu et al., 2016). As reported by Jiang and Xiong (2013), components of soy protein, such as conglycinin and glycinin, contributed to gel formation and improved GS due to the interaction between soy protein and myosin in the protein matrix. This interaction improved the GS of MPGs by activating the catalysis of 7S globulin by MTG (Jang et al., 2016). Because of the presence of globulins in FBPI, the MP structure was changed due to the formation of hydrophobic interactions in this study. These results were supported the interaction of legume proteins, such as FBPI, as substrates for MTG.
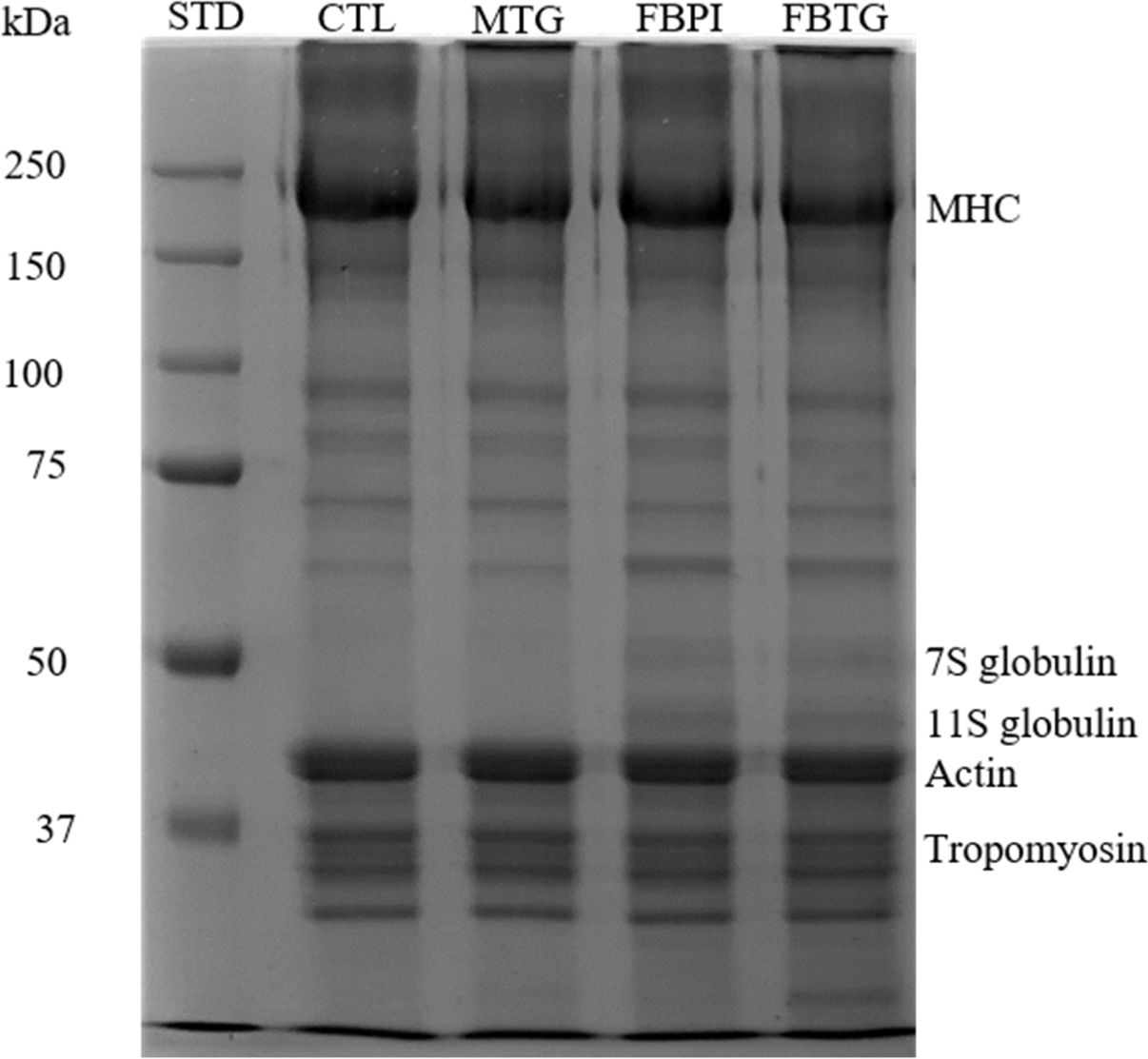
The 3D microstructure of MPGs as affected by the addition of FBPI and MTG alone or in combination are shown in Fig. 3. The surface of CTL was not flatter than other treatments despite its relatively rougher appearance. MPGs with FBPI alone had a flatter surface and more homogenous particles compared to CTL. These micrographs showed that the incorporation of FBPI into MPGs affected the 3D matrix of the protein network by causing structural changes such as the presence of globulins and hydrophobic interaction. The surfaces of MPGs with MTG were different from those without MTG. Protein particles of those with MTG were coagulated and bound to each other tightly, forming fewer voids than CTL or MPG with FBPI alone. The microstructure of FBTG might be formed by stronger binding resulting in a flatter surface than treatment with MTG alone. It is considered that these differences are due to the action of MTG on the combination of FBPI and MTG. Several studies reported that the incorporation of legume proteins, such as peanut (Lin et al., 2019), red bean (Jang et al., 2016), and pea (Borderías et al., 2020), modified to compact surface and decrease the porosity of gels in protein structure. These results suggest that many legume proteins have the potential to improve the functional properties of gel matrices. The incorporation of MTG to pork MPGs can form a homogenous gel structure and high GS due to the cross-linking between Gln and Lys, as reported by Hong and Chin (2010). In addition, Wu et al. (2016) reported that the globulins contained in kidney bean protein improved the emulsion properties and gelation of chicken MP because pre-heating of kidney bean protein induced the unfolding of the structure of globulins, which was beneficial for MTG-mediated cross-linking. Therefore, the changes of improved functional properties were detected on micrographs of MPGs by the addition FBPI or in combined with MTG.
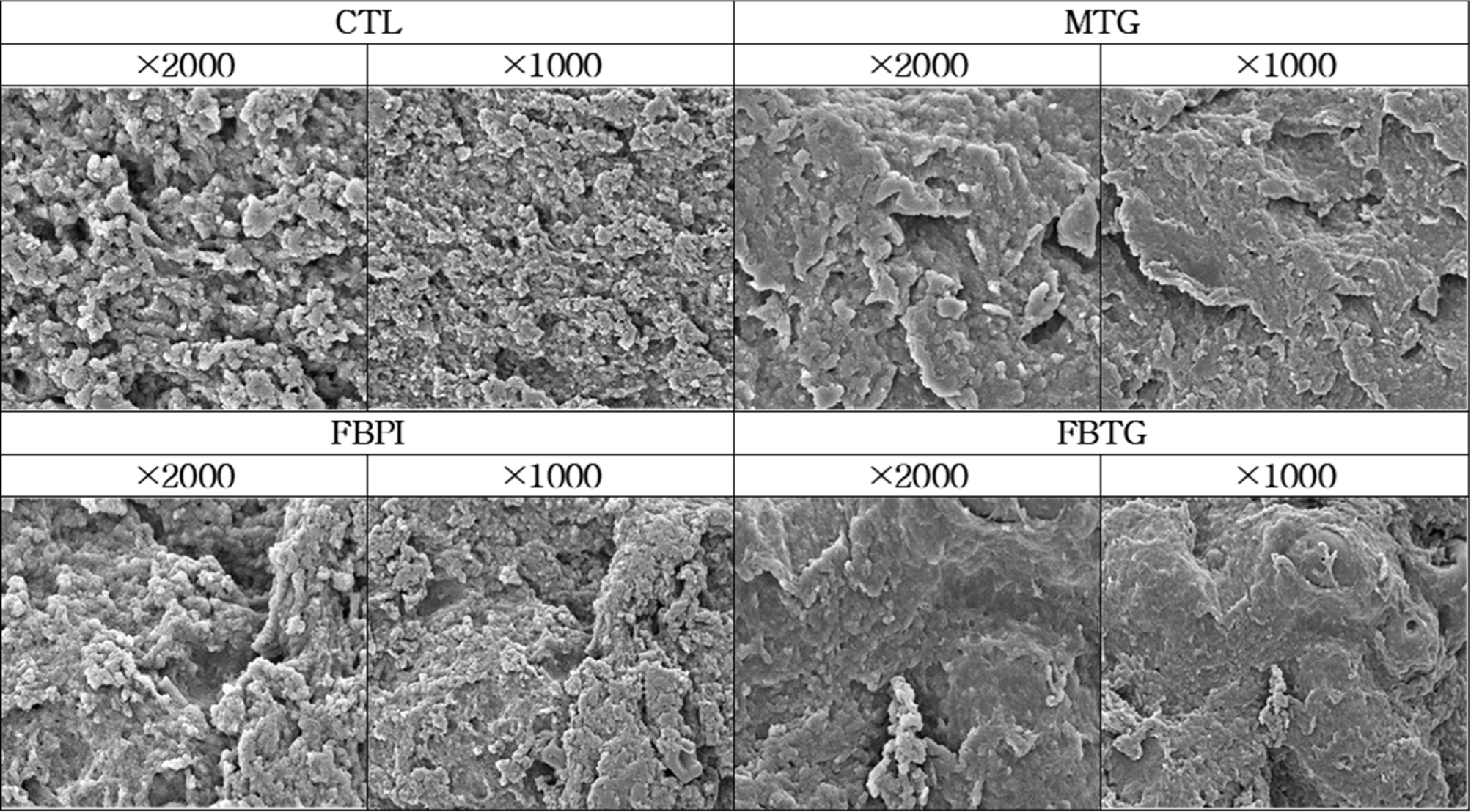
As shown in Table 4, no difference in the pH values of LFMSs was observed among all treatments (p>0.05). These results showed that the different salt levels (CTL vs. REF) and the addition of FBPI and MTG alone or in combination (CTL vs. FBPI, MTG, FBTG) did not affect the pH values. This suggests that the differences in salt levels were not enough to cause a difference in the pH values in this study. Kim and Chin (2019) reported comparable pH values between cooked low-fat pork sausages with 1.0% and 1.5% salt, but these values were lower than those of sausages with 0.5% salt. Lee and Chin (2009) reported that salt concentrations of 0.75%, 1.0%, 1.25%, and 1.5% did not affect the pH values of low-fat pork sausages. It could be that the effect of the salt content on the pH values of pork sausages may be dependent on the other ingredients and their amounts.
Regarding the effect of FBPI, the results were similar to those of Pietrasik and Soladoye (2021), who found no differences in the pH values of cooked low-fat breakfast sausages processed with different pulse fraction, such as pea fiber, starch, flour, and chickpea flour. Moreover, the results concerning the influence of MTG supported those of Choi et al. (2016) that the pH values of semi-dried chicken sausages with 2.0% MTG were not different from semi-dried chicken sausages without MTG. Dimitrakopoulou et al. (2005) studied the effect of salt and MTG levels on the quality characteristics of restructured pork shoulder. They reported that the addition of salt (1.0% and 2.0%) and MTG had no effect on the pH values among all processing and treatment conditions. Therefore, the incorporation of FBPI or MTG did not change the pH values of reduced-salt LFMSs, and those pH values were not different from treatment with regular-level salt (1.5%) and without FBPI and MTG.
The color values of reduced-salt LFMSs with FBPI and MTG are shown in Table 4. There were no differences in all color values (CIE L*, CIE a*, CIE b*) among all treatments (p>0.05). Lee and Chin (2019) reported no differences in the color values of pork model sausages with salt addition levels between 1.0% and 1.5%. Kim and Chin (2019) reported that reduced-salt pork sausage had decreased CIE L* values, especially pork sausage with 0.5% salt compared to 1.5% salt (p<0.05). Depending on the decreased salt content, salt reduction causes changes in the color of meat products, but there were no differences in the color values between LFMSs with 1.0% and 1.5% in the present study. Regarding the influence of added non-meat legume proteins, Lee and Chin (2013a) reported that the color values of pork model sausages formulated with various levels of mung bean flour (0%, 0.3%, 0.6%, 1.2%, and 2.4%) were similar among all treatments. However, the addition of SPI to low-fat pork sausages decreased the CIE a* and increased the CIE b*, as reported by Ahn et al. (1999). As a result, the incorporation of legume proteins in meat products may or may not change the color values, depending on the types and addition levels of proteins and other additives.
When comparing the results of the effect of MTG on the color of LFMSs with previous studies, the findings were similar. To illustrate Lim and Chin (2018) reported that the addition of MTG into low-fat or emulsified model sausages did not affect the color values, regardless of MTG in combination with gluten addition. Furthermore, Choi et al. (2016) reported that the addition of 2.0% MTG into chicken sausage did not affect the color values.
Fig, 4A shows the CL (%) of reduced-salt LFMSs as affected by the addition of FBPI and MTG alone or in combination. The CLs of MPG with MTG alone were similar to those of reduced-salt LFMS with FBPI alone (p>0.05), whereas CTL (without FBPI and MTG) had higher CLs than those of treatments containing FBPI and MTG alone or in combination (p<0.05). In addition, the CLs of reduced-salt LFMS with the combination of FBPI and MTG were lower than those with FBPI alone (p<0.05) and similar to REF (regular-salt; p>0.05). These results indicated that the addition of FBPI could decrease the CLs of LFMSs, and FBTG further exacerbated this effect. Furthermore, despite the lower addition level of salt, the incorporation of both FBPI and MTG into reduced-salt (1.0%) LFMSs could have similar CLs to those with a higher salt level (1.5%).
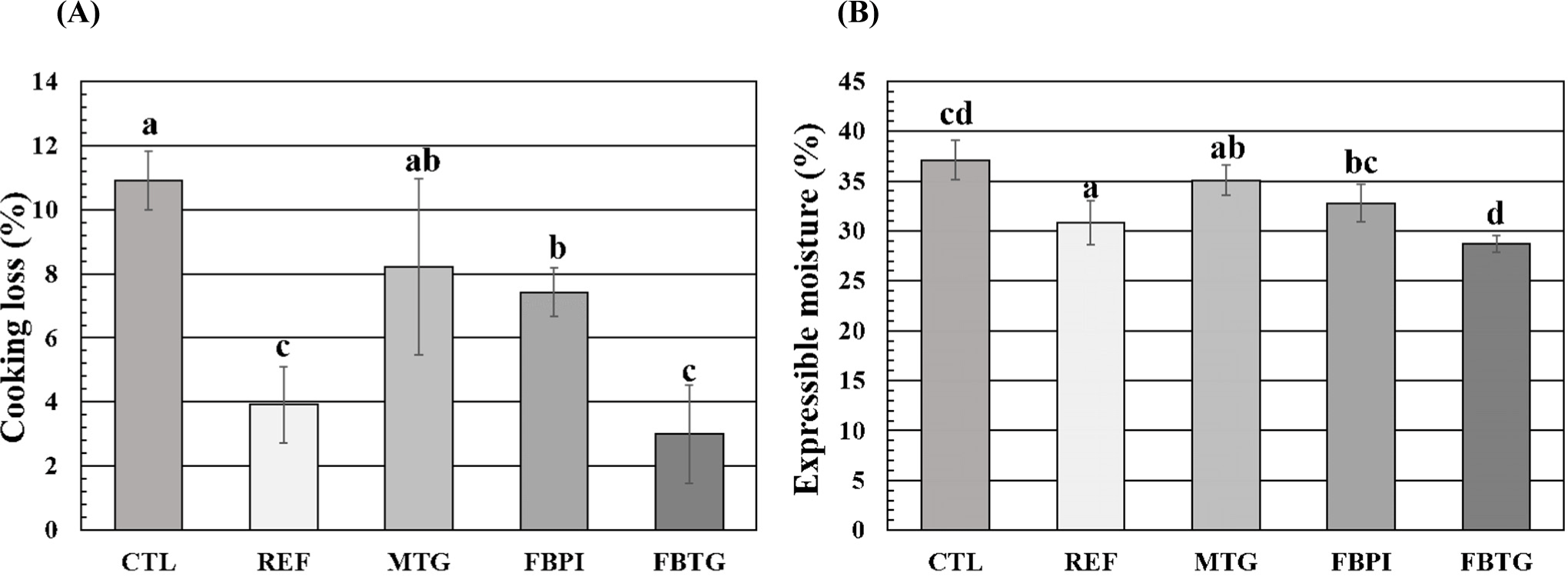
In general, it is known that the reduction of salt might increase the CL of meat products (Ruusunen et al., 2001). It is also reported that legume proteins could improve the CLs of meat products. Beef patties containing PPI (2.5% and 5.0%) as a functional extender (Shen et al., 2022) and buffalo patties formulated with 10% soybean or mung bean flour (Kenawi et al., 2009) had decreased CLs compared to those formulated without extenders. They explained that plant-based proteins had better water and oil retention abilities due to the formation of a cohesive gel matrix and could stabilize meat systems when added at high concentrations.
The addition of MTG alone into MPGs decreased water retention during cooking, but reduced LFMSs with MTG alone had similar CLs to those of CTL in the present study. These results might be due to the fact that the effect of MTG on protein structure was influenced by various factors, such as reaction temperature and time, muscle particle size, and presence of ingredients (Lesiow et al., 2017). Carballo et al. (2006) reported that the negative effect of MTG on WHC of MPs can be explained by an inconsistent development of protein network due to excessive cross-linking. Lee and Chin (2013a) reasoned that the reduction in the CY of low-salt (0.9%) pork model sausages formulated with MTG (1.0%) as a result of their decreased water retention capacity was partially attributed to the absence of a substrate for MTG or the lack of other binding agents as well as excessive cross-linking and aggregation in the protein matrix (Chin et al., 2009). In contrast, the addition of MTG (0.5% and 1.0%) to beef, chicken, and turkey meatballs decreased the CLs of all products, according to Erdem et al. (2020). Some studies reported that MTG causes a decrease in the CL, and therefore, it may be used for inhibiting moisture loss associated with salt reduction during cooking (Pietrasik and Li-Chan, 2002; Pietrasik et al., 2007). Based on these results, the combination of FBPI and MTG might be more appropriate for developing reduced-salt meat products than FBPI or MTG alone.
Fig. 4B shows the EM (%) values of reduced-salt LFMSs. Similar values were found between REF and treatments with FBPI alone or FBTG (p>0.05), and those of CTL and MTG alone (with 1.0% salt) were higher than REF (1.5% salt; p<0.05). It was observed that LFMSs with FBPI were higher water binding ability with a lower EM (p<0.05). The incorporation of MTG into LFMS decreased the EM (%), but samples with MTG alone had similar EMs to CTL. It might be due to the synergistic effect of reduced-salt LFMSs and the action of MTG on FBPI on the WHC. Lee and Chin (2019) reported that sausages made with 1.0% salt had a higher EM (%) than those with 1.5% salt, regardless of the addition of curdlan. When the salt concentration of cooked sausages increased, the WHC increased consequently (Puolanne et al., 2001). The absorption of Cl– ions in salt can move the isoelectric point of myosin to a lower pH, resulting in the improvement of swelling and WHC in an MP system (Hamm, 1986). Because the addition of salt in meat products caused an increase in the WHC, REF had higher functionality than CTL. However, the addition of FBPI into reduced-salt (1.0%) LFMS improved the WHC to that similar to REF. Thus, it suggested that the incorporation of FBPI in LFMS might increase the WHC. Lee and Chin (2022) reported that restructured ham formulated with MTG had lower EM values than without MTG because the addition of MTG can stabilize the water-bound gel of MPs through cross-linking of protein-protein interactions (Gaspar and de Góes-Favoni, 2015). According to Nivala et al. (2021), the addition of MTG into FBPI gels had increased WHC and improved cross-linking ability and foaming properties. As a result, the addition of FBPI alone or in combination with MTG in meat products might have synergistic effects on the WHC.
The textural properties of LFMSs as affected by the addition of FBPI and MTG are shown in Table 5. No differences in the springiness, gumminess, and cohesiveness values were observed among all treatments (p>0.05). However, the hardness and chewiness values of LFMSs were influenced by the different salt level and with or without FBPI and MTG (p<0.05). The hardness values of reduced-salt CTL were the lowest among all treatments (p<0.05). Because salt can improve the functional properties of meat products, the reduction of salt causes a decrease not only in water binding capacity but also in textural properties (Desmond, 2006). Despite the lower salt content (1.0%), LFMSs with FBPI or MTG alone had similar hardness values to those of REF with 1.5% salt (p>0.05). In addition, FBTG had the highest hardness values among all treatments, including REF (p<0.05). The chewiness values of reduced-salt LFMSs with FBPI alone or in combined with MTG were higher than those of CTL and with MTG alone (p>0.05) and similar to those of REF (p<0.05). The addition of FBPI could increase the chewiness values of reduced-salt LFMSs. These results indicated that reduced-salt LFMS with the combination of FBPI and MTG could have improved textural properties.
Several studies have reported the effect of legume proteins in combination with MTG on the textural properties of meat products. For example, Lee and Chin (2013a) concluded that springiness values of low-salt (0.9%) pork model sausages with mung bean flour (0.3%, 1.2%, and 2.4%) were higher than the CTL (1.5% salt). Additionally, Choi and Chin (2020) reported that the incorporation of pea protein concentrate and MTG into LFMSs increased the hardness, springiness, gumminess, and chewiness values compared to the CTL or treatment with SPI. Vegetable proteins are industrially useful as emulsifiers and stabilizers due to their characteristics, which can improve the textural properties and WHC of meat products (Macedo-Silva et al., 2001). The cross-linking of proteins by MTG to form covalent bonds between primary amines, such as Gln and Lys, in protein molecules can enhance the functional and textural properties of meat products (Santhi et al., 2017). Therefore, the combination of FBPI and MTG might be useful for improving the extural properties of reduced-salt meat products.
As shown in Table 5, there were no differences in the moisture, fat, and protein contents among all treatments (p>0.05). Although FBPI has a high protein content (approximately 90%) and can reduce the CLs of LFMSs, it did not affect their proximate composition.
In general, plant-based proteins from legumes are known to have a high protein content (Abete et al., 2009). However, several studies reported that plant-based proteins did not change unexpectedly the proximate composition of meat products due to the low addition level. For example, Vaisey et al. (1975) reported no significant differences in the moisture, fat, and protein contents of ground beef with 0% and 10% legume protein (faba bean concentrate, pea protein, or soy protein as extenders. Additionally, Choi and Chin (2020) concluded that the combination of pea protein (<1.0%) and MTG improved the functional and textural properties of LFMSs without changing the moisture, fat, and protein contents.
Conclusion
The addition of FBPI alone or in combination with MTG into pork MPGs improved the rheological properties, such as CY, GS, and viscosity. The microstructure and SDS-PAGE profile of MPGs illustrated changes in the protein patterns and structure caused by the addition of FBPI or MTG. The addition of FBPI into reduced-salt LFMS could decrease CL and EM, and the combination of FBPI and MTG further exacerbated these effects. The hardness and chewiness values of LFMSs formulated with FBPI increased, and might have synergistic effect when combined with MTG. In conclusion, the addition of FBPI into reduced-salt LFMS improved not only the WHC but also the textural properties, and these effects were further increased when it combined with MTG; FBPI was the potential as a substrate for MTG. In particular, reduced-salt (1.0%) LFMSs containing both FBPI and MTG had similar WHC and textural properties to those with a 1.5% salt level, and could be useful for the development of healthier and functional pork sausage in the meat industry.