Limits and Technological Orientation of Future Protein Resources
Alternative proteins are protein sources manufactured from alternative origins such as plants, microorganisms, edible insects, and cell cultures, instead of animals, to have a form, taste, and texture similar to conventional meat. On the consumer side, these future protein sources are being driven by changing preferences, including curiosity, convenience, personalized nutrition, customized food, animal welfare, and vegetarianism, while in the food industry, they are being recognized as part of environment, social, and governance (ESG) management, as a sustainable development model to meet current needs while meeting the expected needs of future generations. In addition, owing to the rapid growth in population and income worldwide, the demand for meat is increasing rapidly, and is expected to increase by more than 1.4% per year until 2031 (Organisation for Economic Co-operation and Development [OECD] and Food and Agriculture Organization [FAO], 2022). The United Nations has projected that the world's population will reach approximately 9.5 billion by 2050, which will double the demand for animal-derived proteins, including meat, which raise concerns about food security (Henchion et al., 2017). Under these circumstances, the development of future protein resources is expected to reduce the societal need for resource conservation and environmental pollution, as well as meet the diverse needs of people with specific dietary requirements, such as vegetarians. In addition, consumer preferences and interest in diverse protein sources drive the growth of the plant-based protein market as awareness of environmental issues and animal welfare evolve, and changing perceptions of animal protein consumption are raising the need for sustainable future protein production models that meet environmental, social, and economic goals. As the growing demand for future protein sources cannot be met by production in any country alone, future protein resources and related industries, such as, must be considered on a global scale. Currently, protein-related studies and global food companies are engaged in the research and development of alternative proteins as a future protein resource. Many products have already been launched, and the development of related technologies is gradually increasing (Fig. 1). Two of these technologies are worth mentioning: extrusion molding and three-dimensional (3D) printing. Extrusion molding is one of the most commonly adopted technologies for formulating meat analogs, as it organizes proteins under high-temperature conditions with shear forces to mimic the microstructure and texture of muscle tissue and can achieve a certain level of texture similar to that of conventional meat. In the food sector, 3D printing technology can also be used to produce meat analogs as 3D structures by placing and stacking the desired raw materials at specific 3D coordinates using additive manufacturing techniques from programmed 3D model data. This can be used to mimic the delicate muscle fiber structure and intramuscular fatty acids found in conventional meat using various raw materials. Nevertheless, the various Meat analogs products currently available in the market differ significantly from the quality expected by consumers in terms of nutrition and organoleptic properties such as shape, texture, juiciness, color, flavor, and taste compared to conventional meat. Texture, in particular, is influenced by the protein source of the formulation, which is mostly soy protein isolate (SPI) or soy protein concentrate (SPC), except for a few products that are currently on the market. In addition, the extrusion molding process requires initial equipment investment costs due to the industrial machinery required and high utility costs, which increase with the use of high temperature and pressure conditions required during equipment operation. Therefore, the acquisition and operation costs of the equipment are high, making it difficult for meat analogs-producing companies to enter the market. In addition, the textural characteristics of meat analogs, such as uniform stirring and structural alignment of the protein paste, make it difficult to achieve similarity to conventional meat. Currently, most domestic meat analogs products are manufactured using soybeans (Glycine max L.), which has an amino acid score of 100, similar to traditional livestock products such as beef and pork; however, the characteristic fishy and astringent taste of soybeans has a negative impact on consumer preference (Asgar et al., 2010). Various studies have been conducted to solve these problems, and textured vegetable protein (TVP) can be used at the manufacturing stage to improve texture; however, because TVP is mostly imported from overseas, it is necessary to standardize the technology related to the extrusion molding process of TVP. In addition, the formation of crosslinking bonds through interactions between protein molecules can form a fibrous structure, which may improve texture (Flory and Alavi, 2024). To address the off-odor and characteristic fishy taste of soy proteins, it is important to identify and control the interactions between soy flavor compounds and proteins (Saffarionpour, 2024). Research has also been conducted on the addition of iron chlorophyll or heme (HEM) proteins and natural volatile compounds to impart a meat-like flavor (Gerhard, 2020). Consumers, researchers, and governments may have different views on meat analogs from a nutritional perspective; however, considering that the crude protein content and amino acid score of meat analogs may differ from those of conventional meat, depending on the type of raw material, it is necessary to replicate both the quantity and quality of protein, if it is to be used as a future protein source. Nevertheless, meat analogs using plant-based ingredients are still lacking in many aspects and cannot completely replace existing meat. Thus, future protein products similar to conventional meat can only be realized by improving the form, texture, juiciness, color, flavor, and taste to compensate for the deficiencies of existing meat analogs (Schreuders et al., 2021). To overcome the limitations of meat analogs, it is necessary to lay the foundation for manufacturing high-quality meat analogs by incorporating 3D printing technology in the extrusion molding process, which is essential for texture formation and the inclusion of intramuscular fat (used to grade livestock) in meat analogs.
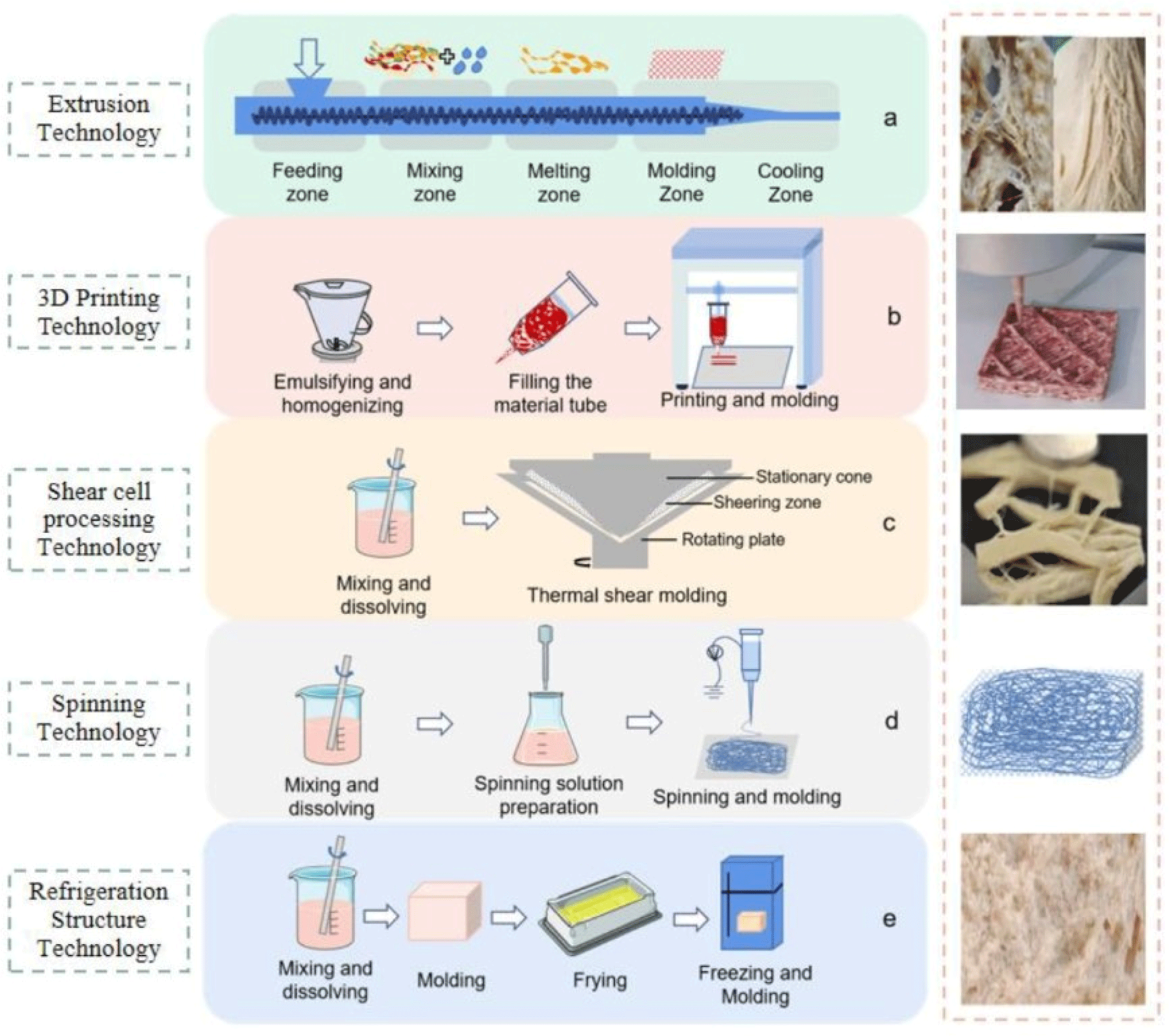
Manufacturing Technologies for Producing Future Protein Resources
Technological approaches to achieve conventional meat-like quality can be broadly categorized into bottom-up and top-down approaches, with different methods exhibiting the characteristics of each approach.
The bottom-up approach involves assembling the structural elements of cells or proteins to achieve a delicate texture similar to that of conventional meat, mimicking the tiny muscle tissues of animal flesh by creating tiny strands of fibrils and assembling them into a single muscle mass. Tissue engineering and mycoprotein production technologies are examples of this approach, as they focus on mimicking the detailed structure of muscle tissue and can effectively mimic the structure of muscle bundles (Samandari et al., 2023); however, they remain limited in terms of scalability and efficiency (Jang and Lee, 2024).
In contrast, top-down focuses on creating a meat-like fibrous structure, typically through an extrusion process that uses externally applied shear forces and stretching to align the polymeric material into fibers. It involves applying heat, pressure, and force to a plant-based protein raw material to create a mass of fibrillar strands. Unlike bottom-up methods, it does not require the production of foundational fine fibrillar strands. However, although it is highly scalable, its ability to perfectly mimic the hierarchical structure of real muscle fibers is limited (Sun et al., 2022).
Many different types of alternative proteins are produced from bottom-up methods, including cultured meat, 3D printing, mycoproteins, and wet spinning. Cultured meat is a technology that grows animal muscle stem cells in culture outside the body and is a potential alternative to actual edible meat. Stem cells used in cultured meat production can be multiplied many times and require far fewer animals than traditional meat production methods. This is an important way to address animal welfare concerns (Zidarič et al., 2020). However, challenges such as high-cost structure, lack of established mass production systems, and improvement in consumer awareness remain in the commercialization of cultured meat. If these issues are addressed, cultured meat has great potential as a future protein source (Bryant and Barnett, 2018). In this regard, bottom-up alternative proteins technologies could be key to building a sustainable food system.
Additive manufacturing using 3D printing technology can precisely place disparate materials such as muscle fibers and fat layers; thus, it can combined small components in a step-by-step manner to create the final product. This approach can be broadly categorized as bottom-up. Methods have been proposed to produce cultured meat using 3D printing by alternating layers of muscle and fat to more precisely mimic the texture of meat, which is an example of a bottom-up approach (Soleymani et al., 2024). Some studies have used 3D bioprinting to reproduce the intricate marbling patterns of Wagyu beef (Fig. 2; Osaka University, 2021).
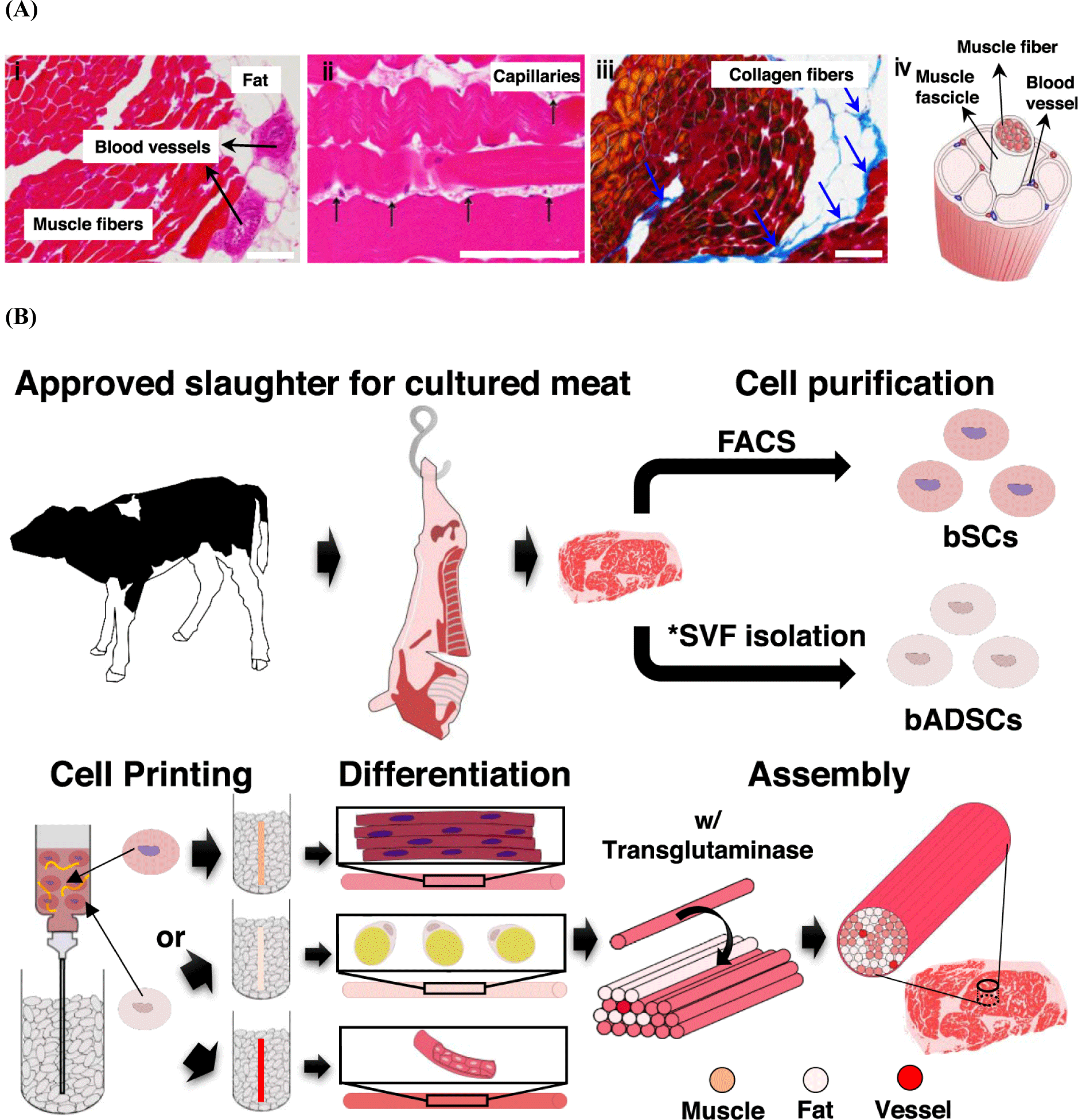
Mycoproteins are produced through a continuous fermentation process in a bioreactor using Fusarium venenatum, a filamentous fungus. This method provides high nutritional content and contains high amounts of essential amino acids that support muscle development. However, studies have reported that the dietary fiber contained in mycoproteins ferments rapidly in the large intestine and may cause gastrointestinal discomfort in some consumers (Derbyshire and Finnigan, 2022). Mycoprotein production was accomplished through fermentation using Fusarium venenatum, as shown in Fig. 3 (Majumder et al., 2024). Wet spinning is a technique used to form fine fiber structures by extruding a protein-containing solution through a spinneret, during which the extruded protein undergoes precipitation, crosslinking, and coagulation to produce thin, uniform fibers (Nagamine et al., 2023). This method is especially advantageous for improving the texture of fibrous foods or alternative proteins and is attracting attention as a fiber formation process using natural proteins. Because the physical properties of fibers can be controlled to achieve unique textures, it is considered a promising technology for the development of meat substitutes. Wet spinning has been successfully used to produce vegetable protein fibers with a texture similar to that of muscle fibers (Joshi et al., 2023). However, wet spinning has certain limitations. First, it involves the use of organic solvents and chemical crosslinkers, which raise concerns regarding food safety and hygiene. These chemicals can cause environmental pollution and raise sustainability concerns (Li et al., 2024). Furthermore, because the fibers formed by wet spinning are generally dependent on the nature of the protein, identifying the correct protein source and solution conditions can be technically challenging. Technical adaptations are essential to meet the stringent hygiene and environmental standards required for protein-spinning processes in the food industry (Tahir et al., 2024).
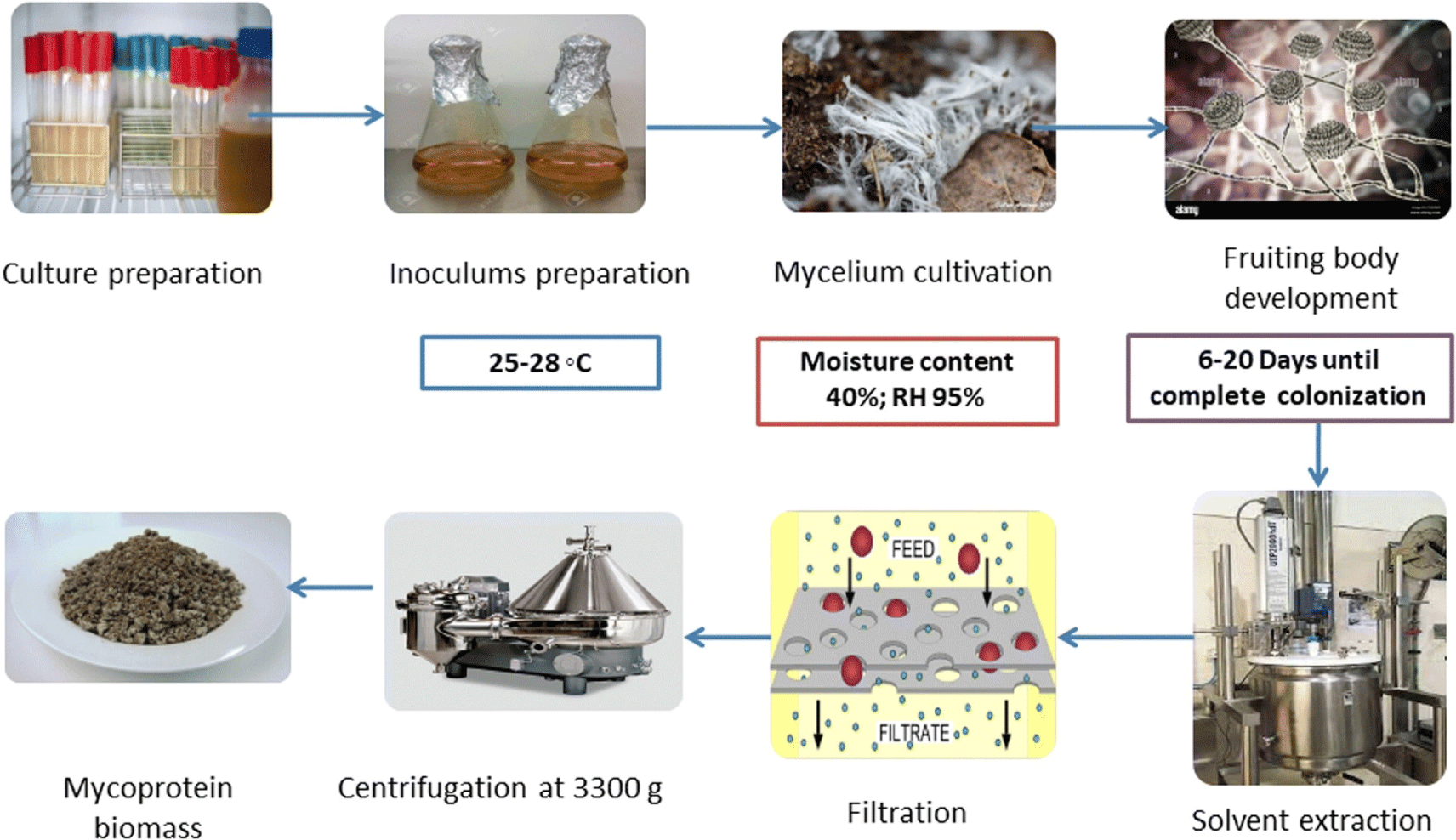
The main technologies for producing alternative proteins from the top-down approach include extrusion, shear cells, protein-hydrocolloid blending, and cryostructuring. Among these, the most widely used commercial method is extrusion, which uses a high-temperature short-time process to induce physical and chemical changes in plant proteins. During this process, the heat and shear forces of the barrel within the extruder reorganize the protein to form a fibrous structure. The outcome of the extrusion process can vary significantly depending on the screw speed, moisture content, processing temperature, nature of the raw material, and addition of polysaccharides. In this process, proteins are heated, hydrated, and melted inside the barrel, and arranged in a fiber structure through the injection port. This creates a texture similar to that of conventional meat; thus, it has been widely used in meat analogs (Table 1; Zhang and Ryu, 2023). Shear cell technology combines shear force and heat to promote fiber formation in proteins, similar to the extrusion process. This is a highly efficient method for aligning proteins into fibers, and is becoming an important topic in research on meat analogs manufacturing. Schematic representations of the extrusion and shear cell processes show their respective fiber structure formation mechanisms (Dobson et al., 2023).
Adapted from Zhang and Ryu (2023) with CC-BY.
In the manufacturing of these meat analogs, different processing techniques have a significant impact on the texture and structure of the final product in different ways. Future meat analogs manufacturing will need to combine the best of both worlds to achieve a more sophisticated and realistic meat-like texture. A hybrid process that combines bottom-up and top-down approaches could consider the use of extrusion molding to replicate the meat-like texture, and 3D printing to apply fat analogs for detailed intramuscular fatness. These new approaches offer the possibility of achieving a better texture and structural similarity to meat. In addition to these technological improvements, improving consumer awareness and emphasizing the environmental benefits to mitigate rejection remain important challenges (De Angelis et al., 2024).
Development of Future Protein Resources Using Extrusion Molding Processes
High- and low-moisture extrusion produces meat analogs with different characteristics, and they have a significant impact on the texture and structure of the final product. High-moisture extrusion is particularly well-suited for forming fibrous structures and allows meat analogs to have a meat-like texture. This is due to the use of a long cooling injection port, which prevents the extrudate from expanding and forms dense fibrous tissue. In contrast, low-moisture extrusion uses relatively short injection holes to form an expanded and porous structure, which is easy to handle even after drying and produces products with a long shelf life (Cho et al., 2023).
Comparing the two processes, high-moisture extrusion provides a texture similar to the fibrous structure of the meat analogs, with high chewability and cutting strength. In contrast, meat analogs produced by low-moisture extrusion are less dense because of their porous structures, resulting in lower elasticity and chewability. These two processes also differ in handling and distribution of products. While high-moisture extrusion can provide an appearance that more closely resembles conventional meat because of the dense fiber structure of the product, the high moisture content makes distribution and storage difficult and shortens the shelf life (Choi and Ryu, 2022). There are several ways to compare the quality of meat analogs produced by high- and low-moisture extrusion.
The degree of organization is assessed by the ratio of the longitudinal cleavage strength to the transverse cleavage strength, which is an indicator of the organized formation of the replacement protein. In general, the organizational degree of replacement proteins created by high-moisture extrusion is higher than that produced by low-moisture extrusion; however, the organizational degree tends to decrease when the moisture content becomes excessively high (Brishti et al., 2020). These results suggest that adjusting of process variables may lead to more diverse organizational patterns in high-moisture extrusion.
Physical property analysis evaluates the physical properties of a product, specifically its hardness, chewability, and cohesion. Products produced by high-moisture extrusion processes have higher elasticity than those produced by low-moisture extrusion; while fiber cohesion is not significantly different, chewability and cutting strength are relatively higher. This is because high-moisture extrusion produces a denser fiber structure (Chen et al., 2023). The tissue residue index quantifies the tissue stability of extruded meat analogs, and the tissue residue index of meat analogs produced by high-moisture extrusion is higher than that produced by low-moisture extrusion. This indicates that high-moisture extrusion forms stronger bonds, allowing the product to maintain its tissue stability even under high temperatures and pressures (Xia et al., 2022).
The soluble nitrogen index is an indicator of protein denaturation and measures the difference in protein solubility before and after the extrusion. The water-soluble nitrogen index of meat analogs produced by high-moisture extrusion is lower than that of proteins produced by low-moisture extrusion. This indicates that the high-moisture extrusion process causes increased protein denaturation, resulting in greater texturization (Samard et al., 2019).
The quality of the meat analogs depends on the characteristics of the extrusion process. By controlling the appropriate process parameters, future proteins with a meat-like texture and structure can be manufactured (Beniwal et al., 2021).
The extrusion process is an important technology for future protein production and many variables must be considered for optimization. The correlation between independent and dependent variables in the extrusion process is highly dependent on the moisture content, barrel temperature, and screw speed (Mateen et al., 2023), each of which plays an important role in protein denaturation and structure formation. Accurate identification and control of process variables are key to the future of meat analogs production, because product quality is highly dependent on proper variable control. Furthermore, these variables can be influenced by different experimental conditions.
The effects of independent and system variables on the dependent variable can be expressed using the following Equation (1):
Where, X represents independent variables, such as feed composition, moisture content, screw speed, and barrel temperature. S represents system variables such as the specific mechanical energy (SME), pressure, and residence time. Y represents dependent variables, such as texture, fibrous structure, expansion ratio, and moisture retention.
This equation represents the interactions between the independent and system variables and their effects on the dependent variables. Changes in each variable significantly influences the texture and physical properties of the final product. Moisture content is an important variable in the extrusion process (Kaur et al., 2022), as it directly affects the denaturation of proteins and starch. Moisture acts as a solvent, plasticizer, and dispersion medium. As moisture content increases, the thermal stability of proteins is enhanced, which facilitates fiber formation (Xia et al., 2023). It also acts as a medium for structural changes in proteins. Therefore, high-moisture extrusion produces fiber-rich, denser, and elastic structures more easily than low-moisture extrusion (Vatansever et al., 2020). The reason for the richer fibers and denser structure in high-moisture extrusion is that the high water content reduces the interaction between proteins, resulting in a smooth and delicate texture. In contrast, low-moisture extrusion produces an expanded porous structure with lower moisture content. This method has advantages such as easier handling and a longer shelf life after drying but tends to result in a less dense and delicate texture.
Therefore, changes in the moisture content have a direct impact on product quality, including juiciness, tenderness, porosity, and appearance.
Barrel temperature is an important factor in the extrusion process that determines the state of the material and the texture of the final product. The extruder barrel consists of four main sections (feeding, mixing, melting, and cooling), where the material undergoes various changes as it moves along the barrel. The temperature of the extruder barrel can be adjusted by section, and temperature changes occurring in each section have a significant effect on protein denaturation. Water and materials are mixed in the feed section; however, owing to low shear and pressure, no significant changes in protein structure occur (Maung, 2020). In the mixing section, the temperature rises from 50°C to 80°C, where the high pressure and temperature loosens and breaks down protein chains, a key change that determines the texture of the final product. In the subsequent melting section, the barrel temperature is increased from 150°C to 170°C, where the protein chains are unwound and structural changes occur under high pressure. Under these conditions, non-covalent and covalent disulfide bonds can break down, causing the molecules to lose their original one-dimensional configuration and disintegrate into smaller units as the chain unwind (He et al., 2013). The extrudate is exposed to high pressure and temperature until it is injected into the nozzle, and when the pressure is released during injection, the swelling of the extrudate destroys the fiber structure formed in the high-moisture extrusion molding process.
Therefore, cooling is necessary to manufacture high-moisture replacement proteins before the melt is injected from the injection port. This process results in the formation of a fibrous structure, which determines the physical properties of the final product (Sui et al., 2024).
The screw speed and configuration play important roles in the process of extrusion molding. The speed of the screw significantly affect the residence time of the raw material inside the barrel. Higher screw speeds increase the shear force exerted on the material, which can promote protein deformation and coalescence. However, if the screw speed is set too high, the residence time of the extrudate inside the barrel becomes too short, which can lead to inadequate heating of the extrudate, poor binding of proteins, and poor texture in the final product (Carneiro et al., 2000).
Screw configuration is a crucial factor affecting the mixing efficiency, screw filling degree, melt-flow behavior, residence time distribution, thermomechanical energy input efficiency, and pressure generation. The consist of forward and reverse feed elements and kneading elements, each of which affects the pressure generation and mixing efficiency. The angle, width, and length of the screw are the main variables that control the filling degree of the material and non-mechanical energy input (Zhang et al., 2015).
Screw arrangement is also an important factor, with the correct combination of forward and reverse screws used to optimize the mixing efficiency and energy transfer. In particular, the reverse screw plays an important role in controlling the filling of the extrudate and non-mechanical energy. Cooling inlets play a key role in the formation of the fibrous structures during high-moisture extrusion. The production of alternative high-moisture proteins by extrusion molding is generally performed using twin-screws and co-rotating extruders with a barrel screw and a cooling injection port at the end (Murillo et al., 2018). Furthermore, the temperature and shear stress at the cooling injection port significantly affect the formation of the fiber structure. The temperature of the cooling nozzle affects the appearance and texture of the product, maintaining pressure as the raw material passes through the nozzle, and preventing the product from expanding to maintain its proper shape. During this process, the protein particles are aligned to form layers and fiber structures. The lower the temperature, the narrower the non-fiber structure at the center of the product and the denser the fiber structure.
The shear rate of the cooling injection port is also an important variable because the shear stresses generated in the narrow port area align with the protein particles, which directly contribute to the formation of layers and fibers (Foegeding, 2015). During cross-sectional deformation and cooling, elongation flow and tensile stresses are generated, which are important factors for protein structure formation. The length of the cooling nozzle and cooling rate also affect the texture and cohesion of the final product, with longer cooling nozzles leading to a better texture. Thus, optimizing the cooling injection port is a critical process that determines the fiber formation of the meat analogs and final product quality (Martínez et al., 2007). The specific mechanical energy (SME) represents the total mechanical energy acting on the raw material in the extrusion process and is an important variable for measuring the strength of the process. In high-moisture extrusion, the SME is low owing to the high moisture content, which results in relatively low shear forces. The SME is an important factor that significantly influences the physical properties of the final product (Fang et al., 2014) and plays an important role in protein denaturation and bond formation, which ultimately determines the physical properties of the final product (Zambrano et al., 2022).
High-moisture extrusion has a low non-mechanical energy input owing to its high moisture content. Lower moisture content increases protein concentration, which increases protein-protein interactions, resulting in increased fibrous structure and density, whereas higher moisture content decreases protein concentration, which decreases protein-protein interactions, resulting in decreased fibrous structure and density (Fig. 4; Hu et al., 2025).

Among the process variables of extrusion molding, the moisture content, barrel temperature, screw arrangement, screw speed, and injection port design affect protein denaturation, crosslinking, and gel formation, which determine the quality of the final product. Therefore, the quality of meat analogs can be improved by appropriately controlling these process variables (Fig. 5).
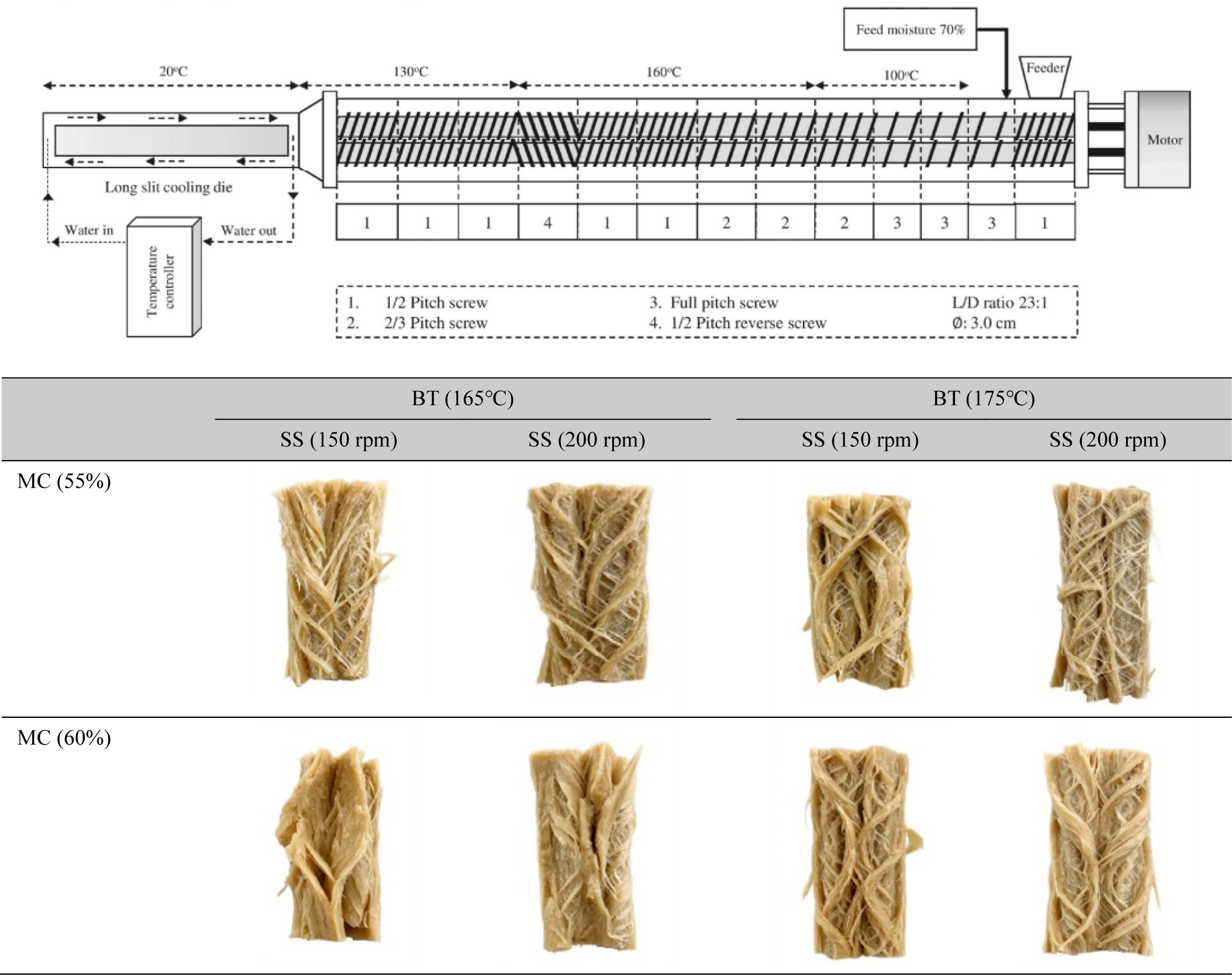
Developing Future Protein Resources Using Three-Dimensional Printing Technology
Using food as a material, 3D printing reshapes the food by placing and stacking the desired material at specified coordinates. These features have made 3D printing a revolutionary method for the food industry, as it allows for the precise stacking of different materials and the reconfiguration of food into desired shapes. The unique additive manufacturing approach to 3D printing, provides a great deal of freedom in designing internal structures such as the arrangement of muscle fibers or the pattern of fat distribution within a muscle. This can play a key role in achieving the meat-like texture and appearance expected by consumers (Kim et al., 2017). meat analogs manufacturing techniques using 3D printing allow for fine control over the placement of muscle fibers as well as the content and composition of nutrients. This can improve the texture of the product as well as its cooking properties, flavor, and appearance. Meat contains complex components, including connective tissue, fiber structure, protein structure, fatty tissue, color, and flavor, which contribute to its inherent organoleptic properties. As meat analogs color formation and flavor enhancement can be considered properties that do not depend on the 3D printing process, 3D printing-related technologies used for meat analogs production can be classified into three types: myofibrillar imitation technology, protein organization technology, and adipose tissue formation. In particular, myofibrillar mimicry focuses on reproducing the fibrous tissue of edible meat, which enhances organoleptic properties such as chewiness. This ensures that the replacement protein has a layered and aligned protein structure similar to that of conventional meat (Dong et al., 2023).
Protein-organizing technologies are also key to 3D printing, allowing proteins to acquire the texture of meat under high temperatures and shear stress. These techniques allow meat analogs to have a unique fibrous structure, giving them a texture similar to that of conventional meat. Adipose tissue formation technology is also an important element in the manufacture of meat analogs, enabling the achievement of intramuscular fat distribution characteristics similar to that of meat, resulting in a more realistic product for consumers. This is particularly relevant for flavor delivery with a focus on simulating fat melting process under heat to release its unique flavor (Shahbazi et al., 2021).
3D printing technology can go beyond simulating fibrous structures to improve the distribution of fat and appearance of meat to maximize consumer experience. It also has the potential to produce personalized products and has applications in the health-food market because different combinations of nutrients can be used to provide customized nutrient formulations and print products with the desired taste and texture. For example, additive technologies to enhance the color and flavor of meat have been introduced as separate processes that can reproduce the natural red color of meat or enhance its flavor (Wen et al., 2023a).
Thus, 3D printing technology is expected to play an important role in the future of the meat analogs industry. Myofibrillar mimicry, adipose tissue formation, and protein organization are important areas of research for improving the texture, taste, and nutrition of meat analogs (Günal-Köroğlu et al., 2024).
Myofibrillar imitation technology is an innovative application of 3D printing that focuses on creating tissue resembling the delicate fiber structure of muscles. This technology works primarily on the principle of layering food materials into 3D structures and shapes, and is designed to improve the organoleptic properties, nutrition, and texture of food products. In the past, 3D printing technologies struggled to recreate the unique texture of muscle fiber arrangements due to their reliance on liquid or pasty materials. Because these materials are limited in their ability to mimic the directionality of real muscles, most 3D-printed protein replacement products focus on mechanical hardness, color, appearance, and basic flavor (Lee et al., 2023). A technique developed to overcome these limitations involves the use of co-axial nozzles, which involves the use of one or more additional nozzle channels to extrude two or more materials simultaneously, thereby enabling a better representation of the muscle fiber structure. In particular, multi-material extrusion with co-axial nozzles can embed a delicate fiber structure within the protein matrix, creating the delicate texture inherent in meat (Fig. 6).
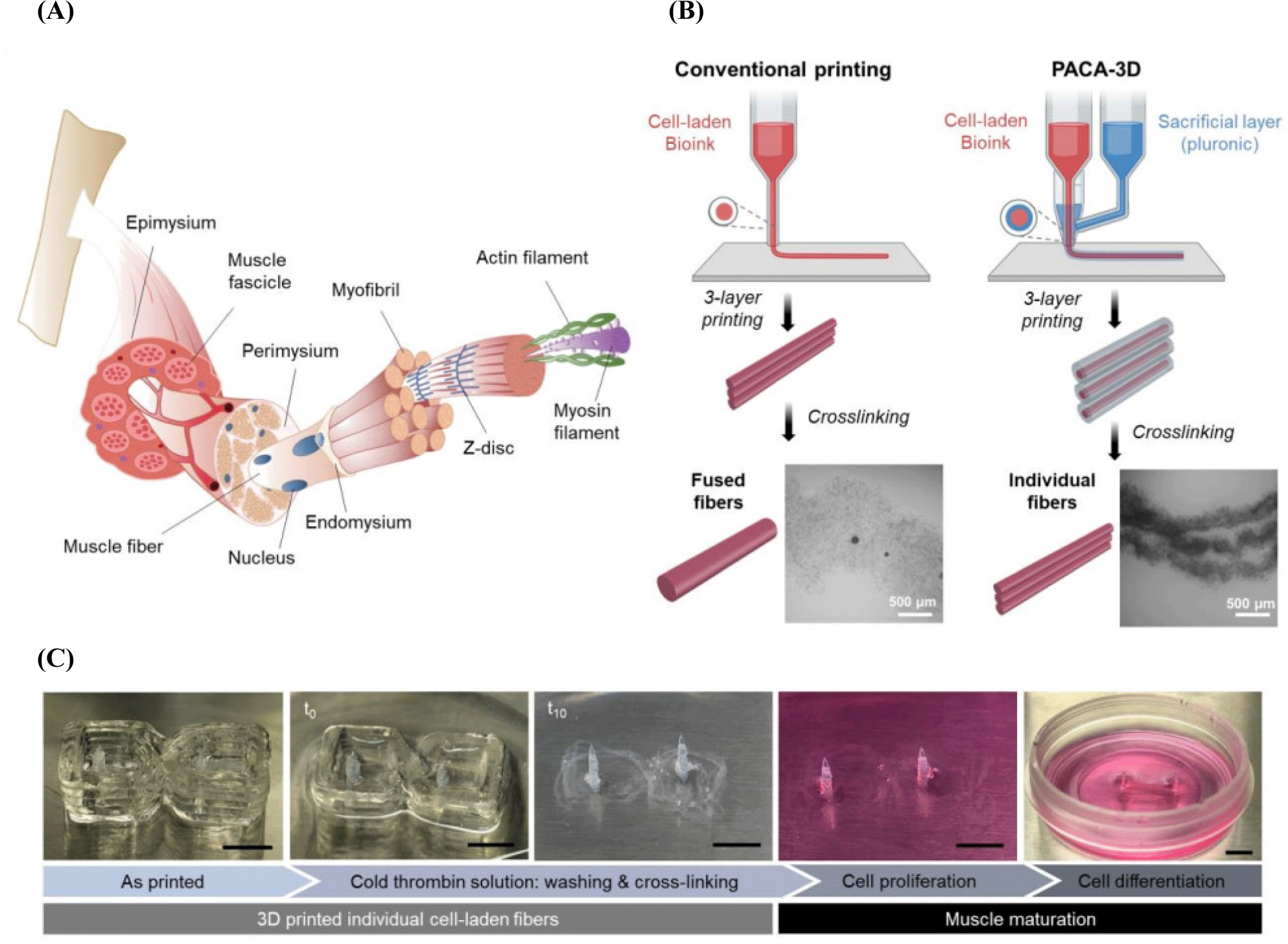
The main materials used are proteins and polysaccharides, which are organized after extrusion through ionic bonding, enzymatic reactions, and thermal denaturation. These processes provide strong fibers similar to that of muscles, which can significantly improve the texture of meat analogs products (Su et al., 2024).
Carrageenan-alginate mixtures form a highly elastic gel when heated and cooled, and additional hardness can be achieved by adding calcium ions. This method is highly effective in mimicking different textures such as beef, pork, and chicken. In addition, the method of arranging myofibrils with co-axial nozzles to express the unique tissue structure can produce cooking properties, product hardness, and elasticity profiles similar to those of conventional meat (Pérez-Mateos et al., 2002).
The 3D printing method using co-axial nozzles will play an important role in meeting the consumer demand for meat analogs products and expanding the diversity of meat analogs products in the future, as it enables variations in the arrangement of muscle fibers and protein sources. In addition, multi-material extrusion using co-axial nozzles can effectively express the fine organization of muscle fibers, opening new possibilities beyond the limits of muscle fiber imitation technology (Ko et al., 2021).
However, there are some challenges associated with this technology. Smaller nozzle diameters allow for finer muscle fibers; however, this comes at the expense of proportionally higher extrusion pressures and lower production efficiency. In addition, multi-material extrusion with co-axial nozzles can incur additional economic costs because two or more materials are extruded into separate channels. To solve these problems, a cartridge-filling technology has recently been developed. By separating the composite material during the process of filling the 3D printer cartridge with the raw material, it is possible to achieve a more detailed representation of the organization of the muscle fibers. As the cartridge-filled material is separated and extruded through the nozzle, different scales of muscle fibers are formed, which can speed up production, even when using relatively large-diameter nozzles (Park et al., 2022).
These advances open new avenues for the production of meat analogs and a wider variety of products quickly and efficiently in the future. Myofibrillar mimicry in the meat analogs industry will play an important role in improving texture, nutrition, and production efficiency (Sharma et al., 2022).
Protein organization is primarily achieved through extrusion molding, a process that uses high temperatures (approximately 130°C) and shear force to denature and organize proteins.
Protein organization at relatively low temperatures is an important research topic in 3D printing. Protein crosslinking via transglutaminase (TG) is the primary technique used in this process. TG is active over a wide range of temperatures, from refrigeration to 50°C, which makes it useful for improving the texture of 3D printed replacement proteins. However, there are some limitations in using TGs. If the TG concentration is too high or the 3D printing time is prolonged, the protein can harden and prevent smooth extrusion, resulting in a rough product surface (Calton et al., 2023). Therefore, it is essential to evaluate the appropriate TG concentration, processing time, and product organization for the final cooking process.
Recent studies have explored the use of enzyme-based or physical organizing techniques in addition to TG. For example, techniques such as sonication or autoclaving can be used in conjunction with TG to further improve the protein structure. These techniques can strengthen protein binding, improve tissue robustness, and potentially be used in various meat analogs products (Ren et al., 2024). Additionally, changes in the texture level depending on the cooking method after 3D printing is also an important challenge. For example, if a 3D printed product is subsequently heat-treated, steamed, or cooked in an air fryer, each method can change the final texture of the product, and it is important to optimize this process. Some studies have suggested optimal cooking conditions to increase water retention and protein aggregation, which play important roles in improving the texture and appearance of meat analogs (Waseem et al., 2024). Therefore, it is important to not only study the concentration, processing time, and cooking method of TG, but also to evaluate the printability and durability of the product. Various variables are being studied to optimize this process, and an efficient process design is still required, especially for increasing the productivity of meat analogs products (Angonese et al., 2022).
The application of fat analogs is a crucial element of 3D-printed meat analogs, offering the technical possibility of mimicking the marbling patterns of raw meat, which is the greatest benefit of producing meat analogs using 3D printing technology.
Marbling indicates the degree of intramuscular fat and is a key quality indicator used in meat grading schemes. Higher levels of marbling are associated with higher levels of organoleptic desirability associated with meat consumption. Animal fat plays an important role in determining the viscosity, texture, taste, flavor, juiciness, and tenderness of meat (Liu et al., 2022). Fat in meat plays an important role in protecting taste, texture, flavor, and juiciness; thus, fat analogs in meat analogs should be the same. When designing fat analogs, various structural enhancers such as methylcellulose, starch, and dietary fiber can be used to produce properties similar to those of real adipose tissue.
However, once organized, fatty analogs are irreversibly molded and unsuitable for extrusion processes because of their poor fluidity. This creates a need for new fat-analog formulations for 3D printing. In particular, in 3D printing, fat analogs affect the final quality of the product through cooling and heating processes, and it is important that the oils flow naturally and remain viscous during cooking. Fat analogs for implementing marbling in 3D printing include carbohydrate-based analogs such as modified starch and dietary fiber, and protein-based analogs such as soy protein and sucrose polyester (Wen et al., 2023b). Studies have utilized gelatinized potato starch in gels filled with inulin emulsions and the addition of potato starch can modify the flow properties, mechanical strength, and printability of fatty analogs. Potato starch affects the structural properties of the inulin matrix; therefore, it can modify the melting behavior of fat analogs during cooking (Oh et al., 2024). In addition, fat analogs based on soy protein, wheat gluten, and cocoa butter can be used in 3D printing to create heat-sensitive cocoa butter similar to the adipose tissue in human meat, as the melting point of cocoa butter is lower than body temperature and exists as a solid at room temperature (Wang and Liu, 2021). With the addition of 15% or more potato starch, soybean and coconut oil-containing fat analogs can attain melting behaviors similar to those of pork and beef fat, respectively (Benković et al., 2023). These techniques ensure that the fat analogs reproduce marbling and have properties that allow the fat to melt and protect the juiciness of the meat analogs during cooking, similar to conventional meat. By placing structured fat analogs in the desired location, 3D printing technology has become a powerful tool for controlling the key components of meat, which could play a crucial role in producing meat analogs products customized to meet consumer demands.
However, balancing production speed and quality is an important challenge for the industrial applications of 3D printing technology. Currently, increasing the production speed by increasing the diameter of the nozzle has been proposed; however, this can have a negative impact on quality details, such as the resolution of muscle fibers. To address this, new extrusion technologies, such as variable nozzles, are being introduced and investigated as a way to increase productivity by enabling one side to be printed simultaneously (Elhadad et al., 2023). Although fat analogs pose a technical challenge in the production of 3D-printed meat analogs, continued research and development will improve results for both food materials and printing processes. 3D printing has the potential to contribute to solving social and environmental problems beyond what is expected, and as technology evolves, a wide variety of products could become available (Tibrewal et al., 2023). The production of meat analogs using 3D printing technology aims to mimic the texture of meat by recombining different components. However, this can be problematic because the original characteristics of the meat are compromised by the grinding of muscle fibers and fat cells, resulting in a ground meat-like texture. This is one of the key challenges in achieving texture and chewability, which are key for meat analogs. Therefore, a technological solution is required to accurately position these fibers and fat cells without crushing them. 3D printing can address these challenges, enabling the reconfiguration of key meat components by precisely placing the desired material in defined coordinates.
However, there are several challenges to the commercial adoption of 3D printing. Food materials used in 3D printing technologies are limited. To date, most studies have been based on additive technologies through extrusion, where paste-like formulations are simply organized through extrusion and cooking. However, this approach has limitations and requires the introduction of additional material-processing techniques. For example, physical techniques such as sonication or high-pressure processing can be combined to promote the binding and organization of proteins (Hussain et al., 2022). Additional research is required before 3D printing of meat analogs can be commercially successful. Nevertheless, this technology has great potential to address social and environmental challenges and as an alternative to sustainable animal agriculture. The possibilities for customized meat analogs production offered by 3D printing technology will gradually expand across industries, and with the introduction of new technological endeavors, there is significant potential for future advances in 3D printing technology (Eswaran et al., 2023).
The Need to Develop Future Animal Resources through the Convergence of Various Technologies
Meat analogs technologies are making significant advances through extrusion and 3D printing and have the potential to further improve the taste and texture expected by consumers. In particular, extrusion, which allows proteins to be organized at high temperatures and pressures to produce a meat-like texture, is becoming an essential technology for achieving a fibrous texture of meat analogs. Therefore, high-moisture extrusion offers significant advantages for myofibrillar imitation, allowing meat analogs to be manufactured with a more natural texture (Miller et al., 2024).
However, there is still an issue of dependence on other countries for key ingredients such as TVP, which can cause disruptions in production if the global supply chain is unstable. This increases the cost of meat analogs and makes it difficult for consumers to reach the expected quality levels. Alternatives to TVP include SPI and SPC. However, these products are limited in their ability to achieve a meat-like texture (Kołodziejczak et al., 2021). Furthermore, although meat analogs are the most prominent development in alternative food products, the quality levels that consumers demand from meat analogs are form, texture, juiciness, color, flavor, taste, and nutritional satisfaction. The current quality level of meat analogs products is limited by the fact that, contrary to consumer expectations, the texture of conventional meat when masticated is similar to ground processed meat. In particular, the quality of some meat analogs products resembles that of ground processed meat when chewed rather than the texture of conventional meat, as consumers expect, which needs to be addressed. While plant-based meat analogs can reduce the dependence on real animal meat consumption and have many benefits in terms of environmental protection and ethical consumption, plant-based proteins can lack the flavor of animal proteins, and vegan products that are 100% plant-based are difficult to create. Therefore, when manufacturing meat analogs, an appropriate balance between plant and animal sources is necessary to satisfy various consumer preferences.
Therefore, it is essential to use flavor enhancers and fat analogs to improve the flavor of meat analogs products. Fat analogs play an important role in improving the flavor of meat analogs products by allowing them to replicate the taste and texture that consumers expect (Fig. 7; Ahmad et al., 2022).
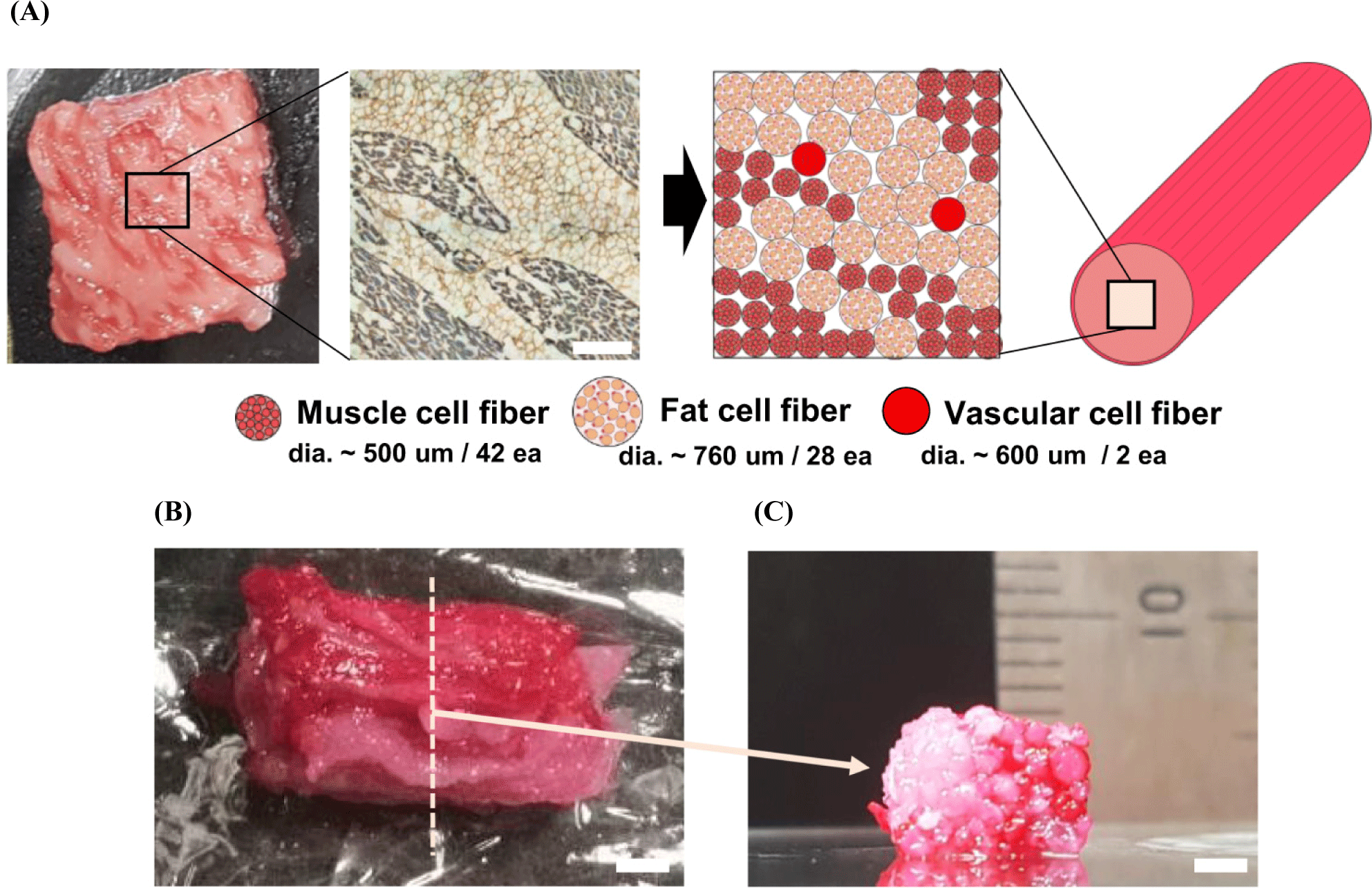
3D printing technology is emerging as an important tool to address these challenges, as it can fine-tune the structure and marbling of meat analogs to achieve organoleptic properties similar to those of meat. In particular, when animal fats are used, the marbling of meat analogs can be reproduced naturally, which is an important factor in increasing consumer acceptance (Park et al., 2023).
3D printing technology can reproduce more than just shapes; it can also provide a sensory experience that mimics that of conventional meat, such as how fat melts away during the cooking process to retain juiciness. This requires innovative technologies such as variable nozzles, which can dramatically speed up production by printing one side at a time, rather than the traditional method of laminating layer-by-layer. Speeding up production while maintaining quality is the key to industrial adoption (Padhiary et al., 2024). Meat analogs have the potential to provide the right blend of plant and animal sources to meet consumer demands. However, successful implementation requires advancements in extrusion molding processes and 3D printing technologies supported by ongoing research and development. These technological innovations are essential for overcoming the organoleptic limitations of meat analogs and for developing better products (Chakravorty and Das, 2024).
In the future, developing a high-moisture extrusion process that can be materialized in various forms will maximize the efficiency of production and distribution of TVP manufactured by specific countries and companies. It is necessary to continue research on using animal fat in TVP along with 3D printing technology to process the marbling of meat analogs that are less palatable to consumers than TVP alone into tissues that resemble conventional meat.