Introduction
Meat is an excellent source of several vital nutrients, including protein, essential amino acids, vitamin B12, zinc, and iron, which are crucial for human health. As the global population grows, meat consumption is also expected to increase correspondingly (Bohrer, 2017). However, the landmark report “Livestock’s Long Shadow” highlighted significant environmental concerns, noting that livestock production accounts for 18% of global greenhouse gas (GHG) emissions, surpassing emissions from transportation (Alexandratos and Bruinsma, 2012; Steinfeld et al., 2006). Additional environmental concerns include the extensive land and water requirements, inefficient resource utilization, and manure-related pollution associated with meat production (van der Weele et al., 2019). Furthermore, growing awareness of climate change has raised concerns about the potential effects on global food sustainability, including impacts on feed and water resources for livestock, as well as direct effects on animal health and reproduction (Godde et al., 2021).
In light of these challenges, there is a rising demand for sustainable dietary alternatives with lower environmental impacts (Bohrer, 2017; Willett et al., 2019). Recently, meat substitutes have garnered attention as a means to reduce the economic and environmental costs of protein production, leading to an increase in their consumption (Jung et al., 2021; Korea Institute of Planning and Evaluation for Technology in Food, Agriculture, and Forestry [IPET], 2022; Park et al., 2020). These substitutes encompass a range of materials, including plant-based proteins, cell culture-based proteins, edible insect proteins, algae-based proteins, and mycoproteins. While research into these alternatives is ongoing and various products are now available on the market (Lee et al., 2020), clear and objective data on their environmental impacts remains scarce. For instance, a report by the Ministry of Environment of Korea indicates that the livestock industry contributes only 1.48% of the country’s total GHG emissions, contrary to the perception that it is a major source of these emissions (Hur et al., 2024). Furthermore, some meat substitutes are subject to greenwashing, which can mislead consumers into overestimating their environmental benefits (Ketelings et al., 2024).
This study investigates the environmental impacts of traditional livestock products compared to alternative protein sources—plant, cell, insect, algae, and fungi—and assesses their potential as sustainable future food options (Fig. 1).
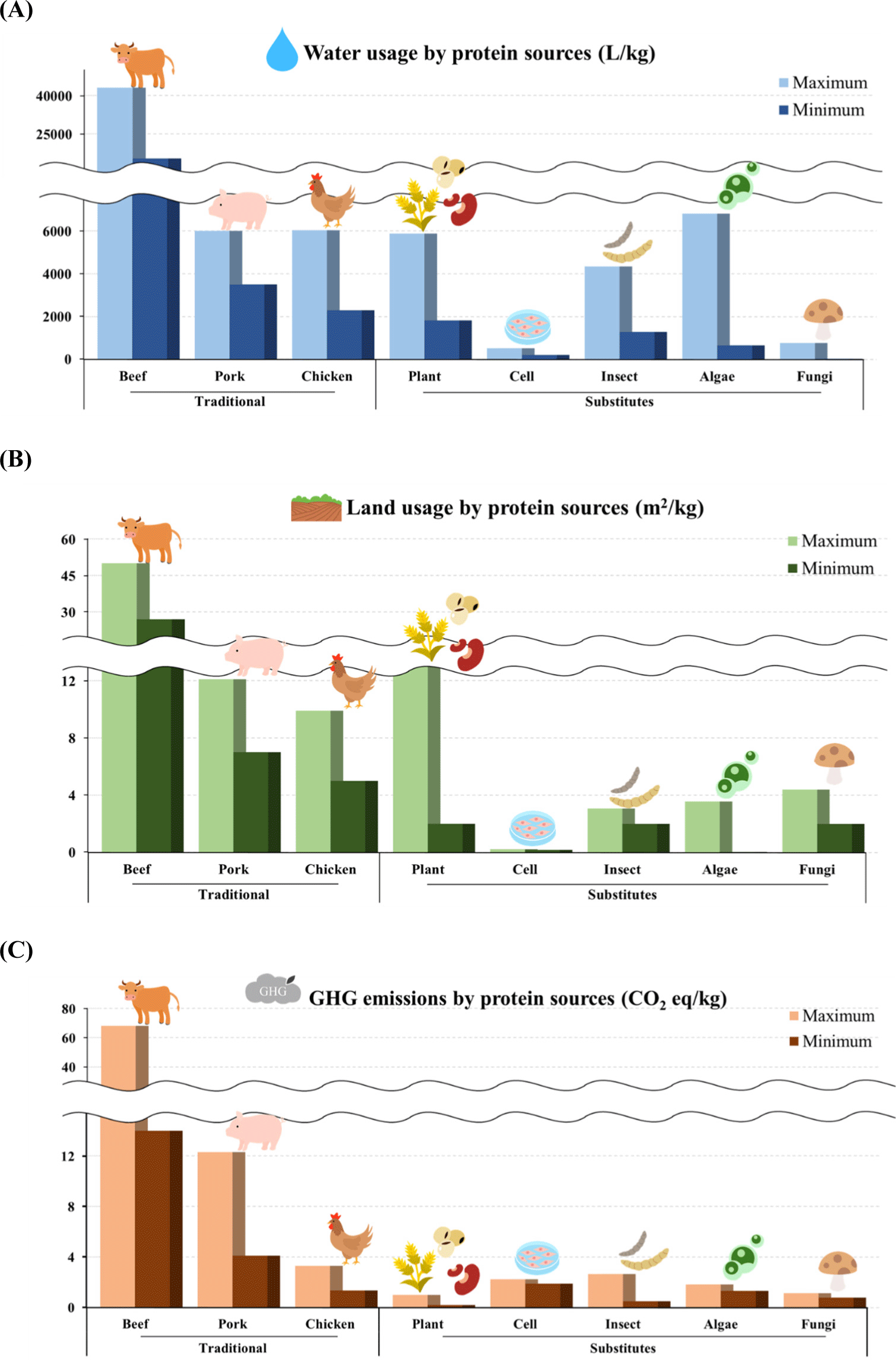
Environmental Impact of Traditional Meat and Alternative Protein Sources
Traditional meat is a vital global food resource, supplying essential nutrients such as protein, amino acids, vitamin B12, zinc, and iron. It is sourced from livestock, including cattle, pigs, chickens, and sheep (Lee et al., 2023). Livestock play a vital role in converting fibrous plant material, which is indigestible to humans, into valuable nutrients (Gerber et al., 2015). Although nutrient composition varies by meat cut, typically, beef contains 13.3%–27.7% protein and 2.41%–28.7% fat, pork has 21.1%–21.6% protein and 3.9%–9.47% fat, and chicken provides 18.6%–22.5% protein and 1.93%–7.92% fat (USDA, 2024). Furthermore, meat not only provides micronutrients such as zinc, iron, calcium, and vitamin B12, but also contains the ‘meat factor’ a substance that helps the absorption of micronutrients (Consalez et al., 2022). Additionally, livestock manure is instrumental in sustaining soil carbon content and fertility due to its richness in organic matter, nitrogen, and phosphorus (Chriki and Hocquette, 2020; Lee and Kim, 2018). The global market for traditional meat reached USD 1.7 trillion in 2023 and is projected to grow at an annual rate of 4.6%, reaching USD 2.6 trillion by 2033 (Precedence Research, 2024).
With increasing meat consumption, the environmental impacts associated with traditional meat production are also increasing. The water used for traditional meat production accounts for approximately one-third of the global agricultural water use. Mekonnen and Hoekstra (2012) reported that the water requirement for producing beef is approximately 15,400 m3/ton, while it is 6,000 m3/ton for pork and 4,300 m3/ton for chicken. Lange and Nakamura (2021) reported that the water requirement for producing 1 kg of beef, pork, and chicken is 22,000–43,000 L/kg, 3,500 L/kg, and 2,300 L/kg, respectively.
Ibidhi et al. (2017) reported that chicken production may require up to 6,030 L/kg of water. Additionally, the land required for raising livestock is substantial, with beef requiring 27–49 m2/kg, pork requiring 8.9–12.1 m2/kg, and chicken requiring 8.1–9.9 m2/kg of land; other studies report similar figures of 30–50 m2/kg for beef, 7–8 m2/kg for pork, and 5–7 m2/kg for chicken (de Vries and de Boer, 2010; Gerber et al., 2015; Souza Filho et al., 2019a). In addition to land use, the production of soybeans and cereals for livestock feed, as well as land conversion for livestock grazing, can result in significant biodiversity loss, particularly in arid regions (Godfray et al., 2018). De Sy et al. (2015) reported that approximately 71% of tropical rainforest conversion in South America was for livestock pastures, while 14% was for growing commercial crops for use as livestock feed. Such extensive land conversion not only impacts global oxygen levels but also diminishes land stability, making it less resilient to environmental changes such as heavy rainfall and drought. Direct and indirect GHG emissions from livestock account for 14.5% of the total anthropogenic GHG emissions worldwide, with cattle raising contributing 65% of this total (Gerber et al., 2013). GHG emissions associated with producing 1 kg of traditional meat are estimated to be 14–39 kg CO2-eq/kg for beef, 4.1–8.9 kg CO2-eq/kg for pork, and 1.35–1.4 kg CO2-eq/kg for chicken (Apostolidis and McLeay, 2016). Some studies report higher values, specifically, 16–68 kg CO2-eq/kg for beef, 6.08–12.3 kg CO2-eq/kg for pork, and 2.6–3.3 kg CO2-eq/kg for chicken (Gerber et al., 2015; Ibidhi et al., 2017; Jeong et al., 2023).
Studies show that GHG emissions from beef production are significantly higher than those from other types of meat. This is primarily due to enteric fermentation in the digestive systems of ruminants like cattle, where gut microbes produce significant amounts of GHGs, such as methane (CH4) and carbon dioxide (CO2), along with additional emissions from manure (Min et al., 2022). GHG emissions from livestock production are calculated by including emissions from the entire production chain, including feed crop cultivation, feed production and transportation, animal rearing, transportation, slaughter, and processing (Hur et al., 2024). These calculation methods differ from those used in other sectors, such as transportation (Hur et al., 2024). For instance, when comparing only direct emissions, Hur et al. (2024) found that agriculture accounts for only 2.9% of the total national GHG emission, with livestock production contributing a mere 1.3%. While data on GHG emissions from ruminants may suggest that they are a major environmental concern, it is important to note that these emissions account for the entire production process, including feed cultivation, feeding, enteric fermentation, and manure management. In contrast, GHG calculations for automobiles focus only on fuel consumption during actual vehicle operation, excluding production processes, making cross-sector comparisons challenging (Park et al., 2022; Steinfeld et al., 2006). Although the environmental soundness of cultured meat production seems promising, the lack of a comprehensive environmental assessment—from the production of materials used to commercial processing—remains a significant challenge for the academic community and stakeholders to validate its sustainability. Therefore, transparency regarding the materials and methods used in both pre- and post-production is essential for providing more accurate estimates of the environmental impact of cultured meat (Hocquette et al., 2024).
Plant-based proteins are extracted from various sources, including peas, soybeans, lentils, lupins, and wheat, and are produced using methods designed to replicate the sensory attributes (e.g., taste and texture) and nutritional profiles of traditional meat (Andreani et al., 2023; Munialo and Vriesekoop, 2023). While protein content varies by crop type, legumes typically contain 20%–40% protein, while grains contain 10%–15% protein on a dry matter (DM) basis. Plant-based protein sources are rich in essential amino acids, unsaturated fatty acids, dietary fiber, vitamins, and minerals, offering several health benefits, such as weight maintenance, reduced high-density lipoprotein levels, and decreased cholesterol levels compared to conventional meat (Ahnen et al., 2019; Erbersdobler et al., 2017; Langyan et al., 2022). Additionally, the growing preference for plant-based proteins is driven by their perceived environmental benefits, such as lower GHG emissions and reduced land and water usage (Aiking, 2011; Coffey et al., 2023; Gibbs and Cappuccio, 2022). Given these nutritional and environmental advantages, the plant-based protein market is projected to grow from $14.2 billion in 2024 to $20.5 billion by 2029, at an annual growth rate of 8.1% (Ahnen et al., 2019; Markets and Markets, 2024a).
Water footprint studies on plant-based protein sources have shown that beans require 2,668 m3/ton of water, while lentils, soybeans, and wheat require 5,874 m3/ton, 2,145 m3/ton, and 1,827 m3/ton, respectively (Derbyshire, 2020; Mekonnen and Gerbens-Leenes, 2020). This water footprint can vary regionally due to factors such as the proportion of irrigated land and the use of chemical fertilizers (Fang et al., 2023). Smetana et al. (2023) reported that 2.0–5.5 m2/kg of land is required to produce the most commonly used plant-based protein sources, including wheat, oats, soybeans, and lupins. Currently, approximately 83% of global agricultural land is used for livestock farming and feed crop production for animal-based proteins. A study conducted in the United States indicated that replacing conventional meat with plant-based proteins could reduce land use by approximately 24% (Eshel et al., 2019; Gibbs and Cappuccio, 2022). Additionally, plant-based protein sources tend to emit fewer GHGs compared to traditional meat. Gustafson (2017) reported that the GHG emissions for the production of legumes, commonly used plant-based protein sources, average 0.27 kg CO2-eq/kg in the United States, which is significantly lower than that of animal-based proteins. In fact, the production of cereals and legumes is known to emit approximately 0.2–1.0 kg CO2-eq/kg, and some companies are promoting plant-based burgers made from soy and pea proteins by highlighting these environmental benefits (Jeong et al., 2023; Smetana et al., 2023; van Vliet et al., 2020).
While plant-based meat alternatives can offer environmental benefits compared to traditional meat, there are also environmental drawbacks that need addressing. Land usage can vary depending on the protein source. For instance, the land requirement for soy production is approximately 0.0014 ha/kg, which is higher than that required for other plant-based protein sources. This makes soy less advantageous in terms of land use compared to traditional meat, except when compared to beef (Derbyshire, 2020). Additionally, organic farming can result in increased GHG emissions. Lee and Choe (2019) found that GHG emissions from organic farming were approximately 20% higher than those from conventional farming, with more than 85% of these emissions coming from fuel, animal manure, and synthetic fertilizers. Other studies also indicate that increasing the yield of plant-based proteins using animal manure and synthetic fertilizers can contribute to GHG emissions (Cellura et al., 2022). Furthermore, Demirdogen et al. (2023) highlighted that both organic farming and monocropping can lead to the use of pesticides and fertilizers, which may cause soil and water pollution and increase susceptibility to pests and diseases. However, these issues can be mitigated through strategies such as mixed cropping with multiple pure seed varieties, efficient water management, and improving energy efficiency using sustainable renewable energy sources like solar power (Boychev, 2022; Faraji, 2011).
Cultured meat is a novel protein substitute produced by inducing the large-scale proliferation of livestock-derived cells and their differentiation into tissues, effectively mimicking the structure and nutritional profile of traditional meat (Bryant and Barnett, 2018; Wikandari et al., 2021). This approach not only ensures a consistent production process but also allows for the customization of nutritional components and taste profiles (Munteanu et al., 2021; Wikandari et al., 2021). According to Markets and Markets, the cultured meat market is projected to grow at a compound annual growth rate (CAGR) of approximately 16.1% over the next five years from 2023, reaching a market size of USD 1.1 billion by 2034 (Markets and Markets, 2024b). Despite its promise as an innovative protein source, full-scale commercialization of cultured meat faces significant challenges, including insufficient safety verification and inadequate regulatory frameworks (Siddiqui et al., 2022). However, its controlled production environment offers several advantages, such as reducing the risk of foodborne illnesses and enabling the engineering of meat with beneficial fatty acids, while avoiding harmful saturated fats. Additionally, cultured meat can be enriched with essential vitamins and minerals during the production process (Bhat et al., 2014; Broucke et al., 2023). To expedite commercialization, ongoing research is focused on optimizing production processes and minimizing environmental impacts, addressing the current limitations (Li et al., 2021; Nikkhah et al., 2023; O’Neill et al., 2021; Rubio et al., 2020; Siddiqui et al., 2022).
Cultured meat shows considerable promise as a sustainable protein source with the potential to reduce the environmental impact of traditional meat production. Its production requires fewer nutrients and energy due to the absence of various bodily organs and also demands less land and water resources (Cho, 2020). Tuomisto and Teixeira de Mattos (2011) reported that producing 1 ton of cultured meat requires 367–521 m3 of water, 190–230 m2 of land, and 26–33 GJ of energy, and results in 1,900–2,240 kg CO2-eq of GHG emissions. Recent studies corroborate these findings, showing values of 95 L/lb of water, 189–232 m2/ton of land, 25.2–31.8 GJ/ton of energy, and 1,891–2,235 kg CO2-eq/ton of GHGs (George, 2020; Rodríguez Escobar et al., 2021), reinforcing the lower environmental impact of cell-cultured protein compared to traditional meat.
Despite having advantages such potential nutritional benefits, reduced resource use, and lower emissions, cultured meat faces significant challenges. High production costs—at least ten times greater than traditional meat protein—and unresolved safety concerns present major hurdles (Garrison et al., 2022; Wikandari et al., 2021). Additionally, the production of cultured meat requires a cell culture medium typically supplemented with fetal bovine serum (FBS) from cattle or serum from horses to efficiently cultivate cells. However, since FBS can only be obtained by slaughtering pregnant cows, the use of FBS makes livestock slaughter unavoidable for the production of cell-cultured protein (Munteanu et al., 2021). To address these ethical concerns, research into serum-free culture media using non-animal materials, such as algae or mushroom extracts, is ongoing. However, the efficiency of these serum substitutes remains limited (Amirvaresi and Ovissipour, 2024). Additionally, some argue that cultured meat production may require more energy than beef production and, if not properly managed, could contribute to eutrophication and water quality degradation due to waste culture media (Haraguchi and Shimizu, 2021; Rubio et al., 2020). Waste culture media from cultured meat production contain compounds rich in nitrogen, phosphorus, and hormones, which, if discharged without proper treatment, can interact with other compounds in the environment, potentially leading to secondary pollution (Chriki and Hocquette, 2020; Haraguchi and Shimizu, 2021; Rubio et al., 2020). Additionally, CO2 can be generated during cultured meat production due to the use of fossil fuels for maintaining optimal culture temperatures. While CH?, a GHG produced by ruminant animals, contributes significantly to GHG emissions, it does not remain in the atmosphere as long as CO2, which accumulates over extended periods (Chriki and Hocquette, 2020). Therefore, there is a possibility that GHG emissions from the rapidly growing cultured meat production industry could have a greater long-term impact on global warming compared to traditional meat production. Consequently, further research is needed to determine whether cultured meat production truly results in lower GHG emissions than traditional meat production and to assess its potential as a solution for achieving carbon neutrality.
Edible insects are gaining attention as a sustainable food source due to their high-quality protein and rich content of polyunsaturated fatty acids. Over 1,900 insect species are used as food worldwide, positioning them as a potential solution for addressing food security and reducing environmental pollution (Hadi and Brightwell, 2021; van Huis et al., 2013). According to Grand View Research, the global insect-based protein market, valued at USD 250 million in 2020, is projected to grow at an average annual rate of 27.4% from 2021 to 2028 (Kati, 2022). Edible insects such as the mealworm (Tenebrio molitor) and house crickets (Acheta domesticus) offer protein contents of approximately 34.2–56.6 g and 39.1–70.0 g per 100 g of DM, respectively, which are comparable to beef, which has a protein content of 54.42–79.18 g per 100 g (Ernawati et al., 2018; Hammer et al., 2023; Kotoura et al., 2012; Miron et al., 2023). In addition to their high protein content, edible insects have excellent nutritional composition. For instance, the black soldier fly larvae (Hermetia illucens) are particularly rich in lysine, an essential amino acid often deficient in staple grains like corn, rice, and wheat, with a content of 8.19 g/100 g, surpassing the recommended value of 4.5 g/100 g (Miron et al., 2023). This suggests that insect-based protein could be a suitable dietary supplement in grain-based diets.
Edible insects have lower environmental impacts and higher production efficiency with minimal resource input compared to traditional meat. They produce over 50% less GHGs and ammonia, require less rearing space, and consume significantly less water compared to traditional livestock, resulting in lower environmental impact (Kim, 2017; Ordoñez-Araque et al., 2022; Ros-Baro et al., 2022). For instance, the water requirement for the production of mealworms and super worms (Zophobas morio) is 4,341 m3/ton (Miglietta et al., 2015), while that for the black soldier fly is 1,293 m3/ton (Galán-Díaz et al., 2024). Additionally, the land requirements for the production of mealworms and black soldier fly larvae are 3.07 m2/kg (Dreyer et al., 2021) and 2 m2/kg, respectively, which is substantially lesser than that required for traditional meat production (Boakye-Yiadom et al., 2022; Dreyer et al., 2021). Oonincx and de Boer (2012) conducted a life cycle assessment (LCA) to evaluate the environmental impact of mealworm production. LCA is a comprehensive tool used to assess the potential environmental impacts and resource use throughout the production cycle, from raw material acquisition to disposal (Finnveden et al., 2009). The LCA results showed that mealworm production has a global warming potential (GWP) 5.55–12.51 times lower than cattle production, and its energy use is 1.02–1.58 times lower, highlighting its reduced environmental impact (Oonincx and de Boer, 2012). These findings align with the GHG emission estimates for mealworms reported by Dreyer et al. (2021) and the GHG emissions data for beef provided by Apostolidis and McLeay (2016). In another study, the GHG emissions of mealworms and black soldier fly larvae were measured at 2 kg CO2-eq/kg and 0.506 kg CO2-eq/kg GHGs, respectively, which are consistent with previous estimates (Galán-Díaz et al., 2024; Lumanlan et al., 2022). Halloran et al. (2017) compared the LCA of crickets and chickens and reported that cricket farming has a lower environmental impact than chicken farming. Conversely, Smetana et al. (2019) found that while black soldier fly larvae have a lower environmental footprint compared to animal-based proteins, they still have a greater environmental impact than plant-based proteins (Halloran et al., 2017). Additionally, as cold-blooded animals, edible insects require less energy to maintain body temperature, resulting in high resource efficiency. This is particularly evident in their feed conversion ratio (FCR): while the FCR for cattle ranges from 2.7 to 8.8, depending on diet and life stage, the FCR for mealworms in the larval stage is 2.2–5.3, and for house crickets in the nymphal stage, it is 1.6–4.5, indicating excellent feed conversion efficiency (Berggren et al., 2019). Moreover, traditional livestock products such as beef, pork, and chicken generate substantial inedible by-products, including bones, internal organs, and leather. In contrast, edible insects produce significantly less waste, with edible parts accounting for 80–90% of their total weight (Ordoñez-Araque et al., 2022). Furthermore, edible insects can convert organic waste into high-quality protein, offering a potential solution for food waste disposal (Lumanlan et al., 2022). For example, when organic waste with low protein content, such as fruits and vegetables (approximately 1% protein), is fed to the black soldier fly larvae, it can be converted into a high-protein food source containing 49% protein (Jucker et al., 2017). This demonstrates how breeding edible insects can contribute to a circular economy by transforming food waste into nutritious and affordable food while addressing food waste management challenges.
Despite the use of controlled rearing environments for edible insects, concerns remain about the potential for insects to escape during rearing. Such escapes could harm biodiversity or disrupt ecosystems. Weissman et al. (2012) highlighted that importing and breeding crickets for commercial purposes could lead to accidental releases, which could cause local biodiversity loss and ecological disturbances. Unlike traditional livestock, escaped insects are nearly impossible to recapture and can easily adapt to new environments, posing significant risks. Therefore, comprehensive research is necessary to select appropriate insect species for edible insect-based protein production, develop systematic rearing systems to prevent accidental releases, and optimize feed and environmental conditions. Additionally, studies assessing the energy use, GHG emissions, and land use associated with various insect species are crucial for objectively evaluating the environmental impact of edible insects.
Algae-based proteins, derived from both multicellular algae (macroalgae), such as kelp and seaweed, and unicellular algae (microalgae), are emerging as sustainable protein sources. These proteins can be cultured in large-scale facilities and obtained through technologies such as ultrasound, pulsed electric fields, and microwaves (Bleakley and Hayes, 2017; Geada et al., 2021). The market size of these algae-based proteins was approximately USD 750 million in 2022 and is projected to reach USD 1.35 billion by 2033, at a CAGR of 5.5% (Future Market Insights, 2023). Among macroalgae, red algae (Rhodophyta) is notable for its high protein content, reaching up to 47% on a DM basis. For instance, Pyropia tenera exhibits protein levels 1.13 times and 1.57 times higher than those of soybeans and peas, respectively (Rawiwan et al., 2022; Suryaningtyas et al., 2023). Furthermore, algae are rich in essential amino acids, carbohydrates, lipids, and minerals, and contain bioactive compounds with antihypertensive and antioxidant effects, making them nutritionally superior (Geada et al., 2021; Thiviya et al., 2022).
Algae-based proteins are gaining attention as alternative proteins due to their high protein content, excellent productivity, and various environmental benefits. Macroalgae have been cultivated in Asia for decades, accounting for 97% of global macroalgae production. Recently, commercial activities have started in Europe, with several ongoing studies focused on cultivation systems (Campbell et al., 2019; Waqas et al., 2024). However, only 25% of studies on the environmental impact of macroalgae production have been conducted in Asia, and there is a notable lack of research on environmental indicators in Europe due to the lack of industrial-scale facilities (Seghetta and Goglio, 2020; Waqas et al., 2024). In contrast, microalgae have been extensively studied for their environmental impacts. For instance, producing 9–12 kg of microalgae through intensive mass cultivation requires only 8,000 liters of water and 32 m2 of land. Other studies estimate water usage at 2 m3/kg, and land usage at 0.04 m2/kg (de Souza Schneider et al., 2018; Herrera et al., 2021; Tzachor et al., 2022). Microalgae also have a minimal impact on water quality and can facilitate large-scale protein production by utilizing food waste as a carbon source (Smetana et al., 2017; Usher et al., 2014). Research indicates that the GHG emission from microalgae production is approximately 1.33 tons CO2-eq/ton, with some studies reporting values of approximately 1.83 kg CO2-eq/kg (de Souza Schneider et al., 2018; Lee et al., 2022). Given the 47% protein content on a DM basis of microalgae, these emissions are relatively low (Suryaningtyas et al., 2023). Furthermore, microalgae are cultivated in CO2-rich environments, allowing them to capture up to 2.48 million tons of CO2 annually during production, which helps mitigate air pollution (Duarte et al., 2017; Geada et al., 2021).
However, algae-based protein production can be energy-intensive, particularly during processing, due to the high moisture content and structural characteristics of the raw material. This can have negative environmental impacts, particularly in the drying stage, which consumes a significant amount of energy. According to Koesling et al. (2021), drying seaweed before protein extraction can utilize various energy sources, including fossil gas, heat, and waste incineration, with CO2 emissions reaching 139 kg CO2-eq/kg when waste incineration is used (Pérez-López et al., 2014; van Oirschot et al., 2017). A proposed solution involves recycling excess energy from incineration, reducing CO2 emissions by more than 10 times to just 12.9 kg CO2-eq/kg of protein produced (Koesling et al., 2021). Additionally, high-pressure homogenization technology, which is used to break down cell walls during protein extraction from algae, is another energy-intensive process, consuming approximately 146.94 kWh of energy per kg of protein produced (Günerken et al., 2015). Water usage also varies significantly depending on the microalgae cultivation process. According to Martins et al. (2018), the water requirement for microalgae grown in photobioreactors is 2.4–6.8 m3/kg, which is higher than that required for cultivation using other methods. Unlike the open pond microalgae cultivation method reported by de Souza Schneider et al. (2018), photobioreactors consume more than 60% of the total water during the operation phase, mainly for electricity and nutrient generation, resulting in significantly higher water usage. While photobioreactors are designed to optimize microalgae production, they still demand substantial amounts of water, electricity, and nutrients, which can amplify their environmental impact. In addition to water, fertilizers are often required to cultivate algae, which can boost microalgae yields but may also contribute to eutrophication. Excess nutrients, such as nitrogen and phosphorus from these fertilizers, contribute to this issue, with phosphate levels reaching 0.034 mg/L in spring and 0.028 mg/L in summer, promoting excessive algal growth and leading to water pollution (Ebrahimzadeh et al., 2021; Jwaideh et al., 2022). Therefore, it is crucial to consider the environmental impact of algae-based protein production and processing and to explore environmentally friendly and sustainable methods.
Overall, algae-based proteins hold promise as sustainable alternative proteins, especially if advancements in resource and energy recycling can reduce energy consumption and CO2 emissions, making algae-based proteins an environmentally friendly option. Therefore, continued research is needed to improve protein extraction methods and maintain clean cultivation environments, ensuring that algae-based proteins emerge as a viable and environmentally friendly protein source for the future.
Mycoprotein, derived from the filamentous fungus Fusarium venenatum, was first discovered in the 1960s. It is commercially produced through fermentation in tanks for approximately 6 weeks. After fermentation, the mycoprotein is collected, dried, heat-treated, cooled, and frozen to achieve a structure similar to traditional meat (Derbyshire and Ayoob, 2019; Park et al., 2023; Sharif et al., 2021). This fungal biomass serves as a high-protein substitute that replicates the texture of traditional meat without actually containing meat. Other fungi used in food fermentation include Aspergillus oryzae, traditionally used in Japan, Rhizopus oligosporus, used in tempeh production, and Neurospora intermedia, used in the Indonesian fermented food ‘Onkom’ (Handoyo and Morita, 2006; Machida et al., 2008; Starzyńska-Janiszewska et al., 2017). According to Future Market Insights (2024), the mycoprotein market is projected to reach USD 720.7 million by 2024 and is expected to grow 1.8 times between 2024 and 2034, demonstrating excellent growth potential. Mycoprotein contains 45% protein on a DM basis, and its Protein Digestibility Corrected Amino Acid Score, which indicates the quality of digestible protein, is 96%–97%, higher than that of soy (91%) or beef (92%; Finnigan et al., 2019; Linder, 2019).
Derbyshire (2020) and Upcraft et al. (2021) reported that water usage in mycoprotein production ranges from 542 m3/ton to 776 m3/ton, which is significantly lower than the water usage associated with beef. Souza Filho et al. (2019a) found that land use for mycoprotein production was 2 m2/kg, while Upcraft et al. (2021) reported a value of 4.390 m2/kg. Studies comparing mycoprotein-based products to beef-based products indicate that mycoprotein uses at least 10 times less water and land than traditional meat (Derbyshire and Finnigan, 2022; Finnigan et al., 2019; Finnigan et al., 2024). The GHGs of mycoprotein were reported to be 0.8 kg CO2-eq/kg in a study by Derbyshire (2020), and approximately 1.14 kg CO2-eq/kg in Microbiology Society (2018), which are significantly lower compared to the emissions from cattle (Shahid et al., 2024). Additionally, research indicates that reusing water from the wheat starch production process during fungal strain cultivation can reduce overall water usage (Souza Filho et al., 2019b).
However, there are contrasting opinions regarding the environmental impact of mycoprotein. Upcraft et al. (2021) reported that water consumption during mycoprotein production could be up to twice as high as for beef. This is because the glucose fermentation process required for the production of F. venenatum A3/5 uses glucose extracted from cellulosic resources, such as straw, rather than agroindustrial residues or mixed food industry waste, which results in higher water consumption (Upcraft et al., 2021). Furthermore, if the quantities of glucose and egg albumin required for mycoprotein formulation are not accurately accounted for, the GWP could reach 5.55–6.15 kg CO2-eq/kg. This is 1.1 to 3.1 times higher than or similar to that of chicken and pork, suggesting that mycoprotein may be less environmentally efficient than conventional meat (Souza Filho et al., 2019a). Research on the environmental impact of mycoprotein production presents mixed opinions regarding its efficiency compared to conventional meat. The current literature includes only a few studies on the LCA of mycoprotein, and there is a lack of research on mycoprotein as a substitute for conventional meat in terms of food demand, land use, and agricultural intensification (Humpenöder et al., 2022). Therefore, to completely understand the environmental impact of mycoproteins, it is essential to continuously research and develop methods to discover fungal strains with superior productivity, increase protein yield, and implement efficient processing methods that minimize environmental pollution.
Comparison among Traditional and Alternative Protein Sources
This study highlights notable differences in water and land use, as well as GHG emissions across various protein sources, based on data from multiple publications (Fig. 1). Beef consistently stands out as the most resource-intensive, requiring the highest amounts of water and land while also being the largest emitter of GHGs. This is likely due to the extended growth and maintenance periods required for cattle compared to other livestock species. In contrast, pork and chicken production show similar levels of water and land use; however, chicken emits less than one-third of the GHGs compared to pork. Among alternative protein sources, both plant-based and algae-based proteins demonstrate water use levels comparable to pork and chicken, yet they significantly outperform traditional proteins in terms of land use and GHG emissions. While alternative proteins are often promoted as more environmentally friendly, insect protein sources surprisingly emit more GHGs than many alternatives, including chicken. Similarly, mycoprotein has higher GHG emissions than chicken, though its water and land use are relatively low. Notably, cultured meat consistently scores low across all parameters; thus, it may be an ideal protein source from an environmental perspective.
However, despite these promising observations, laboratory-grown protein sources, such as cultured meat, algae, and mycoprotein, require further analysis to confirm their low environmental impact. The use of laboratory materials, equipment, and facilities, and the production of media components must be fully accounted for in LCAs to obtain more accurate data. Overall, alternative protein production appears to be a more environmentally sustainable option compared to traditional meat production.
Although alternative protein production promises a more sustainable solution to food insecurity and global warming, enhancing the adoption and consumption of these technologies and products is essential. For example, a survey focused on alternative meat consumer acceptance among New Zealanders revealed that insect-based and cultured meats were less favored compared to plant-based and traditional meats (Hamlin et al., 2022). This finding aligns with the results presented in a systematic review on consumer acceptance of alternative proteins, which identified insect-based proteins as having the lowest acceptability, followed by cultured meat (Onwezen et al., 2021). Furthermore, alternative protein acceptability is influenced by factors such as food neophobia, social norms, familiarity, taste, health motives, and feelings of disgust. A recent study on alternative meat acceptance conducted by Etter et al. (2024) revealed that familiarity with protein sources (e.g., potato, lentil, chickpea, and pea) is key to increasing alternative meat acceptability. It was also recommended that efforts should focus on improving the quality of alternative meats rather than discouraging the consumption of traditional meat. Improving the acceptability of alternative meats could enhance consumption and preference, thereby facilitating the transition towards greener food products.
Conclusion
Based on the reviewed articles and data, alternative protein sources hold significant potential for reducing environmental impact compared to traditional meat. While current assessments suggest that these proteins have a lower environmental footprint, much of the available data on water, land use, and GHG emissions are based on assumptions rather than empirical evidence. This is likely due to the relatively small scale of the alternative protein market to date. In light of this limitation, comparisons between alternative and traditional protein production regarding their environmental impact should be limited to fully accounted production stages. However, excluding evidence outside this scope may significantly distort estimates of key environmental variables (e.g., land use, water consumption, energy use, and GHG emissions). Therefore, employing an evidence-based approach is essential for accurately comparing the environmental impact and sustainability of these protein sources, thereby preventing misinformation among stakeholders and consumers. Additionally, advancements in greener technologies within both traditional and alternative protein industries will be critical to reducing their environmental impact and fostering a more sustainable food production system. Furthermore, enhancing the acceptability of alternative meat products could hasten the adaptation to alternative protein sources and technologies which could influence shifting towards environmentally sustainable food production systems.