Introduction
Gelatin is an inducible protein derived from fibrous protein collagen contained in animal bone, skin, and connective tissues (Karim and Bhat, 2009). In the food industry, it has been extensively used as a gelling agent and stabilizer in various processed foods, along with the advantages of excellent biocompatibility, high biodegradability, and less antigenicity (Gomez-Guillen et al., 2011; Liu et al., 2015). Edible grade gelatin products are commercially obtained from bovine hide and porcine skin, through acidic- or alkalic-treatments (Karim and Bhat, 2009). However, social issues, regarding the risk of bovine spongiform encephalopathy and foot-and-mouth disease, have aroused the suspicions about the safety of bovine hide and porcine skin consumption (Abedinia et al., 2017). In addition, religious dietary restrictions have been recognized as one of the reasons for searching for an alternative gelatin source (Gomez-Guillen et al., 2011). In this regard, several previous studies have been performed to characterize gelatins derived from fish (skin and fin) and poultry (skin and feet) by-products and to determine their practical availability as an alternative gelatin source (Gomez-Guillen et al., 2011; Karim and Bhat, 2009).
In meat products, gelatin as a non-meat protein is practically used to improve cooking yield and textural properties (Lee and Chin, 2016). It has been known that the positive impacts of gelatin addition into meat products are mostly based on its gel-forming ability and water-binding capacity (Gomez-Guillen et al., 2011). However, inconsistent efficacies of gelatin on meat products have been reported occasionally in previous studies conducted with different gelatins and addition levels (Jridi et al., 2015; Lee and Chin, 2016; Yeo et al., 2014). In fact, the negative impact of gelatin addition on water-holding capacity of emulsified sausage has been observed due to the melting out of gelatin during thermal process (Lee and Chin, 2016). In general, the functional effects of non-meat proteins on processing characteristics of meat products, such as milk proteins (whey and casein proteins) and plant proteins originated from soybean, legume, and oilseed, are determined by their own functional properties (e.g. gel-forming ability, water/oil binding capacities, protein solubility, and emulsifying capacity) and their interaction with myofibrillar proteins (Asgar et al., 2010; Sun and Holley, 2011). However, in most previous studies, the effect of gelatin on meat products has been largely understood based on only functional properties of gelatin (Jridi et al., 2015; Park et al., 2016).
Heat-induced gelation of myofibrillar proteins plays an important role in forming desirable water-holding capacity and texture of meat products (Sun and Holley, 2011). However, few studies previously investigated an interaction between myofibrillar protein and gelatin and corresponding impacts on its gel properties. According to Yang et al. (2007), the addition of porcine skin gelatin into porcine myofibrillar protein gels could decrease denaturation temperature of myosin. As a result, this would contribute to increased elasticity and viscosity of heat-induced porcine myofibrillar protein gels. In contrast, Brewer et al. (2005) have indicated that porcine skin gelatin may have negative impacts on the gelation of porcine myofibrillar proteins, since those two proteins have different mechanisms for their gelation without interaction. Thus, it would be necessary to clearly elucidate the interaction between myofibrillar proteins and gelatin under equivalent conditions to evaluate the availability of various gelatins as a protein additive.
Therefore, the objectives of this study were to determine the interaction between porcine myofibrillar proteins and various gelatins (bovine hide, porcine skin, fish skin, and duck skin) and to evaluate the physicochemical and textural properties of heat-induced porcine myofibrillar protein-gelatin gels.
Materials and Methods
Six boneless pork loins (M. longissimus dorsi thoracis et lumborum) for isolating myofibrillar proteins were purchased from a local market at 24 h postmortem. Commercial gelatin powders, such as bovine hide gelatin (gel strength: 280 bloom, particular size: 5–15 mesh, Hangzhou Qunli Gelatin Chemical Co. Ltd.), porcine skin gelatin (gel strength: 280 bloom, particular size: 5–15 mesh, Hangzhou Qunli Gelatin Chemical Co. Ltd.), and fish skin gelatin (gel strength: 250 bloom, particular size: 5–15 mesh, Hangzhou Qunli Gelatin Chemical Co. Ltd.), were purchased. Duck skin gelatin powder (gel strength: 210 bloom), which was extracted through acid treatment (pH 4), super-heated steam extraction (150°C for 10 min), and lyophilization, was kindly provided by the Korea Food Research Institute.
Porcine myofibrillar protein was isolated from pork loins according to the procedure of Wu et al. (2009) with minor modification. Briefly, trimmed and chopped pork loin was homogenized with 4 volumes of isolating buffer {0.1 M NaCl, 10 mM sodium phosphate (pH 7.0), 2 mM MgCl2, 1 mM ethylene glycol-bis (2-aminoethylether)-N,N,N′,N′-tetraacetic acid (EGTA)} for 3 min. The homogenate was centrifuged at 3,000×g for 15 min (4°C). The supernatant was discarded, and the residual pellets were resuspended in the isolating buffer (4 volumes). This process was repeated three times. The final pellets were homogenized again with 4 volumes of 0.1 M NaCl solution, and a few drops of 1 N HCl were added to adjust the pH of the myofibrillar protein suspension to pH 6.2 and centrifuged again at the same conditions above. The supernatant was discarded, and the protein concentration of myofibrillar proteins was determined by the biuret reaction method (Gornall et al., 1949). The isolated porcine myofibrillar protein was stored in a 4°C refrigerator and used within 48 h after isolation. In total, three independent batches were performed.
The porcine myofibrillar protein was suspended with dilution buffer {50 mM sodium phosphate (pH 6.2), 0.6 M NaCl} in 50 mL conical tubes (Wu et al., 2009), in which the protein concentration of all treatments was equally fixed as 60 mg/mL. For gelatin treatments, the ratio of porcine myofibrillar protein and each gelatin was 9:1 at the same protein concentration, which is one of the optimal mixing ratios for improving gel strength (Lan, 1994). The porcine myofibrillar protein-gelatin mixtures were heated from 10 to 75°C in an 80°C water bath, in which the heating rate was 2°C/min. After cooling in a 4°C refrigerator for 12 h, the heat-induced porcine myofibrillar protein-gelatin gels were blotted with paper towels, weighed, and used to evaluate gel properties.
The pH value of heat-induced porcine myofibrillar protein-gelatin gels was measured in triplicate using an electric pH meter equipped with an insert-type pH probe (HI 99163, Hanna Instruments Inc., Woonsocket, RI).
The instrumental color of heat-induced porcine myofibrillar protein-gelatin gels (cross-sections) was measured using a colorimeter (CR-400, Minolta, Osaka, Japan) equipped with an 8 mm observer (diameter). The setting for the illuminant was D65 source. Calibration of the instrument was conducted with a calibration tile (CIE L*: +93.01, CIE a*: −0.25, CIE b*: +3.50), according to the manufacturer’s manual. The CIE L*, a*, and b* values were taken from five random locations, and chroma (color intensity) was calculated as follows: chroma, (American Meat Science Association , 2012).
The cooking yield of heat-induced porcine myofibrillar protein-gelatin gels was calculated as a percentage difference between initial and cooked weights.
The water-holding capacity of heat-induced porcine myofibrillar protein-gelatin gels was determined in triplicate by the centrifugal method described by Xia et al. (2010) with minor modification. Briefly, 3 g of intact gel were placed in a 50 mL conical tube, and the tube was centrifuged at 3,000×g for 15 min (4°C). The water-holding capacity was calculated as follows: centrifugal weight loss (%) = [{the weight before centrifuge (g) − the weight after centrifuge (g)}/the weight before centrifuge (g)]×100. A high value of centrifugal weight loss would indicate poor water-holding capacity.
Texture profile analysis of heat-induced porcine myofibrillar protein-gelatin gels was conducted using a texture analyzer (CT3, Brookfield Engineering Laboratories, INC. Middleboro, MA). The gels were equilibrated to room temperature at 25°C for 3 h, and four samples (cylinder shape of 2.5 cm height and 2 cm diameter) were taken from the central portion of each gel. A twice compression cycle test (70% compression of the original sample height) was performed with a cylinder probe (diameter in 4 cm). Sample deformation curves were obtained with a 50 kg maximum load cell and the analysis condition were as follows: pre-test speed 1.0 mm/s, post-test speed 5.0 mm/s, test speed 2.0 mm/s. Value for hardness (kg), springiness (ratio), cohesiveness, gumminess (kg), and chewiness (kg) were determined according to the calculating method of Bourne (1978).
SDS-PAGE of porcine myofibrillar protein-gelatin mixtures was performed by the Laemmli method (1970), using 12% running gels and 5% stacking gels. Gelatin samples and protein mixtures were diluted with 25 mM sodium phosphate buffer (pH 7.2) containing 1% sodium dodecyl sulfate (SDS) and 3.5 M urea, in which the protein concentration of samples was 50 mg/mL. The sample mixtures were heated at 85°C for 1 h and centrifuged at 8,500×g for 5 min to discard insoluble residues. The samples were mixed with 5× Laemmli sample buffer (312.5 mM Tris-HCl (pH 6.8), 50% glycerol, 5% SDS, 5% β-mercaptoethanol, 0.05% bromophenol blue, EBA-1052, Elpisbiotech, Daejeon, Korea) at the ratio of 4:1 and heated at 100°C for 5 min. Fifteen microliters of each sample were loaded at 100 V for 2 h. The loaded gel was stained with Coomassie Brilliant Blue R250 (B7920, Sigma, USA) and destained in methanol:distilled water:acetic acid (50:40:10). The separated protein bands were identified by comparison with a standard protein marker (pre-stained DokDo-MARK, EBM-1032, Elpisbiotech, Daejeon, Korea).
The thermal denaturation temperature of porcine myofibrillar protein-gelatin mixtures was measured in triplicate using a DSC machine (Q200, TA Instrument, New Castle, NJ). Approximately 15 mg of protein mixtures were accurately weighed into an aluminum pan, and an empty pan was used as a reference. Samples were heated from 5 to 95°C at a constant rate of 10°C/min (Doerscher et al., 2004). The temperature of endothermic peaks was analyzed using a Universal Analysis 2000 program (TA Instruments, New Castle, NJ) according to the manufacturer’s guidelines.
The experimental design was a completely randomized block design with three independent batches. Analysis of variance was performed on the measured variables, such as pH, color, cooking yield, centrifugal weight loss, TPA, and DSC results, using one-way ANOVA procedure of the SPSS program (SPSS Inc., Chicago, IL, USA). Duncan’s multiple range test was used to determine significant differences between means (p<0.05).
Results and Discussion
The pH value and water-holding capacity of heat-induced porcine myofibrillar protein-gelatin gels are shown in Table 1. The pH value of porcine myofibrillar protein-gelatin gels ranged from 5.71 to 6.01, which was unaffected by gelatin addition (p>0.05). In general, it has been reported that the addition of gelatin has little impact on the pH value of meat products (Lee and Chin, 2016; Yeo et al., 2014), since acid- or alkali-extracted gelatin is necessarily neutralized to pH 5.0–7.0. While the duck feet gelatin (pH 3.04) used in this study was not neutralized, no significant effect on the pH value of heat-induced porcine myofibrillar protein gel was observed.
The water-holding capacity of porcine myofibrillar protein-gelatin gels was determined through the measurement of cooking yield and centrifugal weight loss (Table 1). The addition of gelatin led to a decrease in cooking yield of heat-induced porcine myofibrillar protein gels (p<0.05). In particular, duck skin gelatin treatment (68.97%) exhibited a significantly lower cooking yield compared to control (87.27%). Further, the addition of gelatin slightly decreased centrifugal weight loss of heat-induced porcine myofibrillar protein gels (p<0.05), indicating improved water-holding capacity. In particular, bovine hide gelatin and porcine skin gelatin treatments (7.10% and 7.79%, respectively) showed significantly lower centrifugal weight loss than control (11.08%). These results could indicate no positive interaction between gelatin and porcine myofibrillar proteins during heating process, but gelatin addition may be effective in retaining moisture within myofibrillar protein heat-induced gels. Moreover, the decreased cooking yield of duck skin treatment was likely due to relatively low pH toward isoelectric point of myofibrillar protein, although the pH difference between treatments was not significant.
Previous studies have reported inconsistent results regarding the impact of gelatin on water-holding capacity of meat products, especially on cooking yield or loss. According to Yeo et al. (2014), the addition of duck feet gelatin (2–3 g/100 g), as a fat replacer, could decrease the cooking loss of emulsion sausages. In contrast, Lee and Chin (2016) reported that the cooking loss of model sausages increased by increasing the added level of porcine skin gelatin (0.5–1.5 g/100 g) and explained that the observed phenomenon might be due to “melting out” of gelatin during the thermal process. In addition, they suggested that the positive impact of gelatin on water-holding capacity could be influenced by fat content in meat products, since gelatin may interact with lipid molecules, as an emulsifying agent (Lee and Chin, 2016). In a heat-induced myofibrillar protein gel (nearly fat-free system), thus, gelatin may have no positive effect for minimizing water release during the thermal process. However, gelatin gel after heating could contribute to improved water-holding capacity, thereby entrapping moisture within its gel matrix structure.
The color characteristics of heat-induced porcine myofibrillar protein-gelatin gels are shown in Table 2. CIE L* (lightness) and b* (yellowness) of the gels were affected by gelatin addition (p<0.05); however, there were no significant differences in CIE a* (redness) and chroma (color intensity). All gelatin treatments exhibited more light and yellow color than control, in which duck skin gelatin treatment showed the highest lightness and yellowness among all treatments (p<0.05). Similarly, Yeo et al. (2014) noted that the addition of duck feet gelatin to emulsion sausages increased yellowness but did not affect redness. The color of gelatin is mainly influenced by raw materials and extraction conditions, such as pH, temperature, and time (Aykin-Dincer et al., 2017). Commercial bovine hide gelatin and porcine skin gelatin are generally colorless, whereas the gelatins obtained from poultry by-products (e.g. skin and feet) show slightly whitish-yellow color (Park et al., 2013). According to Ockerman and Hansen (1988), however, the color of gelatin may have no influence on its functional properties.
The textural properties of heat-induced porcine myofibrillar protein-gelatin gels are shown in Table 3. The addition of gelatin significantly decreased hardness, cohesiveness, gumminess, and chewiness of heat-induced porcine myofibrillar gels. However, there was no significant difference in springiness of the gels. Similarly, Brewer et al. (2005) noted that the addition of gelatin could cause negative impacts on myofibrillar protein gelation, resulting in a decrease in gel strength. They suggested that those two proteins, myofibrillar protein and gelatin, may have compatible mechanisms on gelation without interaction, which could in turn weaken the gel strength (Brewer et al., 2005). In this study, the gelatin type had little to no impacts on the textural properties of heat-induced porcine myofibrillar protein gels. Among gelatin treatments, the only significant difference in hardness was found between fish and duck skin gelatin treatments, while duck skin gelatin had a lower bloom value than fish skin gelatin. This result was likely due to the relatively high amount of released water in duck skin gelatin treatment, as shown by a decrease in cooking yield.
Protein pattern of porcine myofibrillar protein-gelatin mixtures was analyzed to determine the non-thermal interaction of those two proteins using SDS-PAGE in the presence of β-mercaptoethanol (Fig. 1). All gelatin samples (gelatin only) showed two evident bands at 100–140 kDa, which could be expected as α1- and α2-chains (∼100 kDa), major subunits of gelatin (Kim et al., 2014). Bovine hide gelatin, porcine skin gelatin, and fish skin gelatin additionally presented dimers and trimers of α-chains, namely β-chain (∼200 kDa) and γ-chain (> 240 kDa). However, such dimers and trimers were not observed in duck skin gelatin, although many protein bands at diverse molecular weights below 100 kDa (mainly ∼40 kDa and ∼30 kDa) were found. Those proteins at lower molecular weights compared to α-chains were probably derived from α-chains of duck skin gelatin, due to the relatively higher extracting temperature of super-heated steam compared to the commercial heating temperature for gelatin extraction. Similarly, Gomez-Guillen et al. (2002) indicated that the high temperature for gelatin extraction could result in a fragmentation of α-chains. When compared to the protein pattern of porcine myofibrillar proteins, porcine myofibrillar protein-gelatin mixtures exhibited no differences in protein pattern and intensity. This result may indicate no interaction between porcine myofibrillar proteins and gelatin under non-thermal conditions. Furthermore, no changes in band intensity at the molecular weights of gelatin components (e.g. α-chains at 100–140 kDa and β-chain at 200 kDa) were found. According to Kim et al. (2014), the addition of 3%–6% duck feet gelatin into duck muscle increased the band intensity at the molecular weights of α- and β-chains. The different result of the current study was likely due to the relatively low ratio of gelatin to porcine myofibrillar protein.
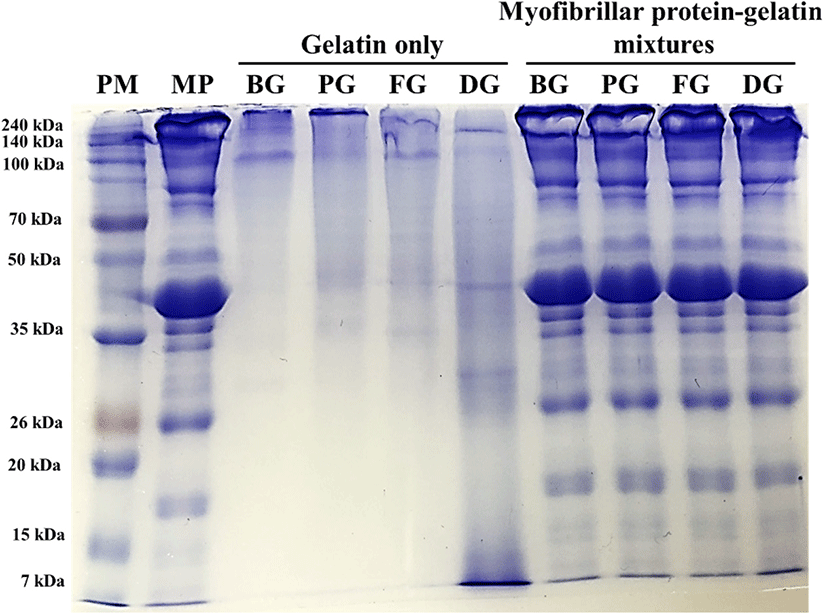
The DSC thermograms and endothermic peak temperature of porcine myofibrillar protein-gelatin mixtures are shown in Fig. 2 and Table 4, respectively. Pure porcine myofibrillar proteins (control) showed two evident endothermic peaks at 57.22°C (peak 2) and 68.64°C (peak 3), which could be expected as the thermal denaturation temperature of myosin and actin (Liu et al., 2000). The addition of gelatin resulted in the appearance of a new endothermic peak at 30°C–40°C (Fig. 2). The highest temperature of endothermic peak 1 was observed in the fish skin gelatin treatment, although there was no significant difference (Table 4). The appearance of endothermic peak 1 was likely due to gelatin melting. Thus, the different temperature of endothermic peak 1 could be associated with thermal properties of each gelatin, since the melting temperature of gelatin is species-specific (Gomez-Guillen et al., 2011). Moreover, the addition of gelatin altered the temperature of endothermic peak 2 (p<0.05; Table 4), whereas a similar temperature of endothermic peak 3 was observed among all treatments regardless of gelatin addition (p>0.05). The porcine myofibrillar protein-gelatin mixtures showed significantly lower temperatures at endothermic peak 2 compared to pure porcine myofibrillar protein (control). Previously, Yang et al. (2007) reported that the addition of gelatin decreased the denaturation temperature of pure myosin from 52°C to 47.5°C and suggested that the decreased denaturation temperature of myosin might be due to the interaction between myosin and gelatin. The previous suggestion was consistent with our observation. In particular, the noticeable decrease in peak 2 temperature might be related to the presence of many fragmented peptides, since the fragmentation of gelatin subunits could improve the solubility and/or interaction with other biological molecules (Gomez-Guillen et al., 2002).
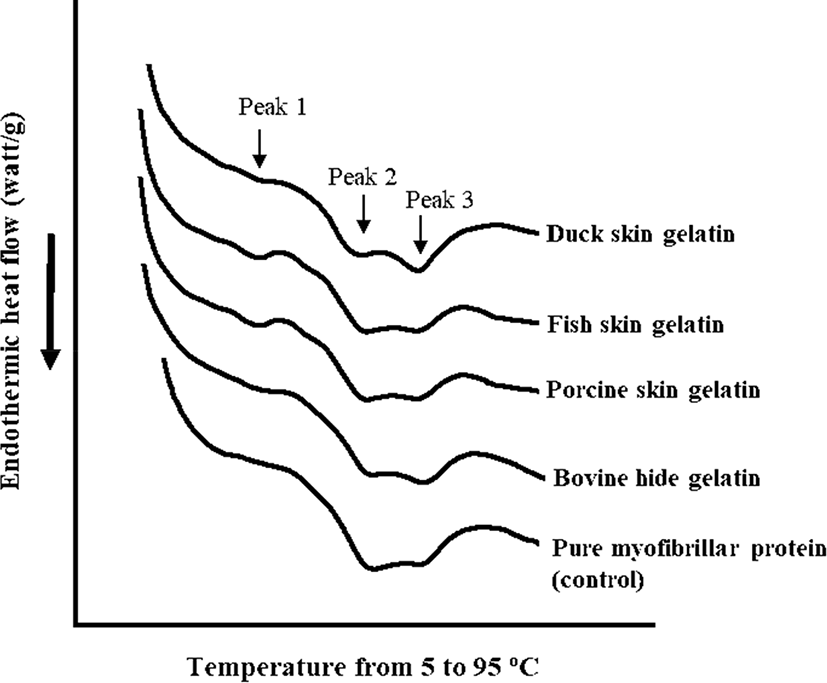
Conclusion
The results of this study show that the addition of gelatin at low concentration (6 mg/mL) could attenuate water-holding capacity and textural properties of heat-induced porcine myofibrillar protein gel. In particular, the inadequate cooking yield and hardness were found in duck skin gelatin and fish skin gelatin treatments, respectively. While the result for thermal properties determined by DSC could provide limited information on weak thermally induced interactions between myosin and gelatin, it would be insufficient to override the negative impacts. Thus, it could be suggested that well-known positive impacts of gelatin on quality characteristics of meat products may be mainly related to the functional properties of gelatin per se, rather than its interaction with myofibrillar proteins. In addition, results of the current study indicate that mammalian gelatins extracted from bovine hide and porcine skin could be effective in improving water-holding capacity of heat-induced porcine myofibrillar gel.