Introduction
Hypertension is strongly associated with substantial cardiovascular disease risks, such as heart attack and stroke (Khalesi et al., 2014). Recent findings revealed that the increasing global prevalence of hypertension is a pressing health concern worldwide, affecting nearly one in two Americans (Ritchey et al., 2018). Furthermore, an estimated 1.3 billion adults are affected by hypertension worldwide (NCD Risk Factor Collaboration, 2021). Nitrovasodilators, such as organic nitrates and sodium nitroprusside, have proven effective in managing hypertensive crises because nitric oxide (NO) is a vasodilator directly linked to blood pressure regulation (Bryan, 2022). Therefore, NO is pivotal in regulating hypertension, and the reduction of NO bioavailability, otherwise known as endothelial dysfunction, is a characteristic feature of arterial hypertension (Hermann et al., 2006).
Additionally, inadequate NO production impairs blood flow regulation and circulation, subsequently increasing inflammation, oxidative stress, and immune dysfunction (Cervantes-Gracia et al., 2020). Vascular smooth muscle cells (VSMCs), which constitute the vascular wall, are crucial for regulating hypertension due to their plastic and dynamic features (Touyz et al., 2018). Extensive studies have reported that the Rho-associated kinase (ROCK) signaling pathway and its downstream effectors, such as the myosin light chain (MLC), promote inflammation and remodeling in VSMCs, resulting in the pathogenesis of cardiovascular disease (Zhang et al., 2015). Moreover, enhanced ROCK signaling via MLC phosphorylation contributes to VSMC contraction (Ma et al., 2023).
Probiotic lactobacilli, including Lactiplantibacillus plantarum, have emerged as a novel therapeutic approach for regulating blood pressure. For instance, L. plantarum supplementation significantly reduced blood pressure in hypertensive patients (Sharafedtinov et al., 2013). Additional studies have indicated that probiotic supplementation had positive effects on lowering blood pressure (Dong et al., 2013; He et al., 2017; Khalesi et al., 2014). Malik et al. (2018) demonstrated that L. plantarum 299v supplementation improved vascular endothelial function and decreased systemic inflammation in patients with coronary artery disease. Furthermore, conditioned media derived from L. acidophilus exhibited anti-apoptotic and anti-inflammatory activities in human umbilical vein endothelial cells (HUVECs) treated with lipopolysaccharide (LPS; Kalani et al., 2016). However, although extensive clinical trials reported positive effects on lowering blood pressure, the cellular mechanism by which probiotic lactobacilli accomplishes this attenuation is not well documented. Thus, this study investigated the impact of spray-dried L. plantarum K79 (LpK79) on NO production and elucidated its intracellular signaling pathway in HUVECs. In addition, the signaling pathway in vascular function and hypertension was also examined.
Materials and Methods
Spray-dried LpK79 was kindly provided from Novalacto (Daejeon, Korea). Briefly, pasteurized skim milk containing glucose was aseptically inoculated with LpK79 and incubated at 37°C until pH reached 4.2. Next, the bacterial culture was subjected to heat treatment at 85°C for 20 min, cooled at 50°C, and subsequently underwent spray drying.
HUVECs were purchased from the American Type Culture Collection (ATCC; Manassas, VA, USA) and maintained in Kaighn’s modification of Ham’s F-12 medium (Gibco, Burlington, ON, Canada) supplemented with 10% fetal bovine serum (Gibco) and antibiotics (HyClone, Logan, UT, USA). The cells were seeded on a 96-well culture plate and treated with various concentrations of LpK79 (0, 2.5, 5, and 10 mg/mL) at 37°C for 24 and 48 h. After obtaining spent culture supernatants, NO production was measured using a nitrate/nitrite fluorometric assay kit (Cayman Chemical, Ann Arbor, MI, USA).
HUVECs were seeded onto a 100 mm tissue culture dish and incubated at 37°C for two days. Then, cells were treated with various concentrations of LpK79 (0, 2.5, 5, and 10 mg/mL) at 37°C for 2 h, and cell lysates were obtained using a lysis buffer. In a separate experiment, VSMCs were purchased from ATCC and maintained in Kaighn’s modification of Ham’s F-12 medium (Gibco) under culture conditions as previously described. VSMCs were seeded and incubated at 37°C for two days. Afterward, cells were treated with the different concentrations of LpK79 (0, 2.5, 5, and 10 mg/mL) at 37°C for 2 h, and cell lysates were obtained using a lysis buffer to determine ROCK2 and MLC activation. Lysate concentrations were determined using a bicinchonic acid protein assay to perform western blot analysis. Equal volumes of lysate samples were loaded on a 10% sodium dodecyl sulfate-polyacrylamide gel electrophoresis, electro-transferred onto polyvinylidene difluoride membranes (Millipore, Bedford, MA, USA), and blocked with 5% skim milk. Next, the membranes were incubated with primary antibodies specific for phosphoinositide 3-kinase (PI3K), phosphorylated PI3K, Akt, phosphorylated Akt, endothelial nitric oxide synthase (eNOS), phosphorylated eNOS, MLC, phosphorylated MLC, ROCK2 (Cell Signaling Technology, Danvers, MA, USA), or β-actin (Santa Cruz Biotechnology, Santa Cruz, CA, USA) overnight at 4°C. After washing the membranes with Tris-buffered saline containing 0.1% Tween 20, membranes were incubated with horseradish peroxidase-conjugated IgG at room temperature for 2 h. Immunoreactive bands on the membranes were detected using an enhanced chemiluminescence reagent (Dyne Bio, Seongnam, Korea) and visualized with a C-Digit blot scanner (LI-COR Bioscience, Lincoln, NE, USA).
HUVECs were seeded in a 6-well culture plate and incubated at 37°C for two days. The cells were then stimulated with Escherichia coli LPS (1 μg/mL) with or without LpK79 (0, 2.5, 5, and 10 mg/mL) at 37°C for 3 h. Total RNA was extracted, and complementary DNA (cDNA) was synthesized using random hexamers and M-MLV reverse transcriptase (Promega, Madison, WI, USA). Pro-inflammatory cytokines, interleukin (IL)-6, and IL-8 genes were amplified through polymerase chain reaction (PCR) using the SYBR green real-time PCR master mix (Toyobo, Osaka, Japan) and a StepOnePlusTM Real-Time PCR System (Applied Biosystems, Foster City, CA, USA) according to the following conditions: initial denaturation at 95°C for 10 min, 40 cycles of denaturation at 95°C for 15 s, and annealing/extension at 60°C for 1 min. The specific primers used in this study were as follows: IL-6, forward 5’-TGCAATAACCACCCCTGACC-3’ and reverse 5’-GTGCCCATGCT ACATTTGCC-3’; IL-8, forward 5’-GTGCAGTTTTGCCAAGGAGT-3’ and reverse 5’-CTCTGCACCCAGTTTTCCTT-3’; and GAPDH, forward 5’-AAGGTGAAGGTCGGAGTCAA-3’ and reverse 5’-ATGACAAGCTTCCCGTTCTC-3’. The relative mRNA expression of IL-6 and IL-8 was normalized to that of GAPDH using the 2–ΔΔCT method.
To determine the protein secretion of E. coli LPS-stimulated IL-6 and IL-8 in HUVECs with LpK79, HUVECs were seeded as previously described, and cells were stimulated with E. coli LPS (1 μg/mL) with or without LpK79 (10 mg/mL) at 37° for 24 h. After obtaining supernatants, IL-6 and IL-8 were quantified using enzyme-linked immunosorbent assay (ELISA) kits (R&D Systems, Minneapolis, MN, USA).
Statistical significance between groups was determined through one-way analysis of variance (ANOVA) using IBM SPSS Statistics 23 software (IBM, Armonk, NY, USA) or unpaired two-tailed t-test using GraphPad Prism 5 (GraphPad Software, La Jolla, CA, USA). p<0.05 was considered statistically significant.
Results and Discussion
When HUVECs were incubated with LpK79 at different concentrations (2.5, 5, and 10 mg/mL) for 24 h, LpK79 dose-dependently increased NO production (Fig. 1A). HUVECs were further incubated with LpK79 for 48 h and LpK79 also significantly increased NO production in a dose-dependent manner (Fig. 1B). Previous studies demonstrated that probiotic lactobacilli exert antihypertensive effects in animal models and human clinical trials (Gómez-Guzmán et al., 2015; Jauhiainen et al., 2010; Malik et al., 2018). Our findings corroborate the previous reports that LpK79 promotes NO production in HUVECs, subsequently reducing hypertension. NO released by endothelial cells are critical regulators for vasculature relaxation. Moreover, NO diffused by smooth muscle cells promoted relaxation by increasing cyclic guanosine monophosphate levels (Gu et al., 2020). Although the impact of particular compounds from probiotics on the vascular functions has not been thoroughly explained, a study has demonstrated that short chain fatty acids reduce the blood pressure through G protein-coupled receptor 41, present in vascular endothelial cells (Natarajan et al., 2016). Thus, it may be speculated that metabolic short chain fatty acids derived from probiotics play a role in the regulation of vascular functions.
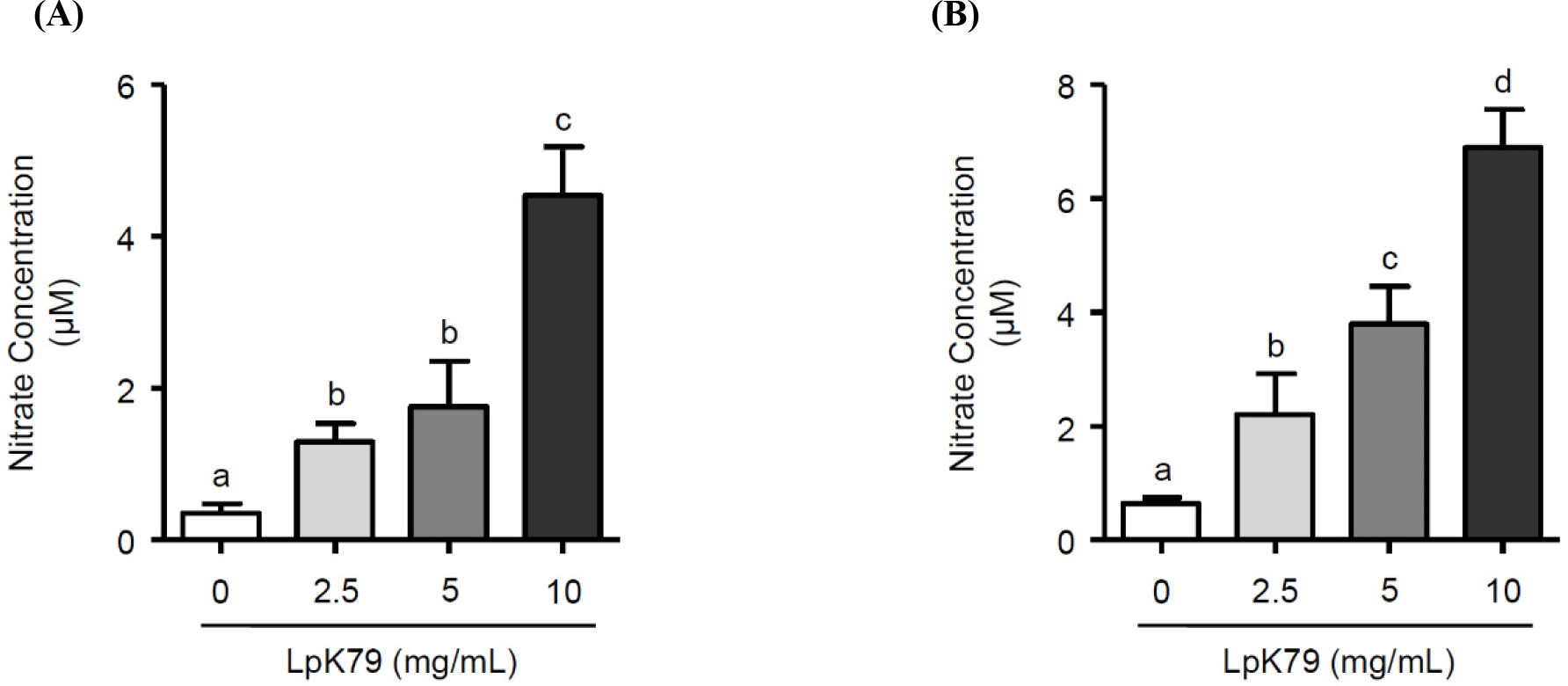
eNOS primarily facilitated NO production in vascular endothelium. Numerous studies discovered that the phosphatidylinositol 3-kinase (PI3K) pathway triggers the activation of serine-threonine protein kinase B (Akt), leading to the direct phosphorylation of eNOS (Ahmad et al., 2018; Gu et al., 2020). HUVECs were exposed to LpK79 at concentrations of 2.5, 5, and 10 mg/mL for 2 h to investigate the impact of LpK79 on PI3K, Akt, and eNOS phosphorylation. As shown in Fig. 2A, LpK79 significantly enhanced PI3K phosphorylation at 5 and 10 mg/mL and Akt phosphorylation at 2.5, 5, and 10 mg/mL. Furthermore, eNOS phosphorylation increased in the presence of LpK79. A recent report determined that while the probiotic Bifidobacterium longum ZL0210 did not substantially affect PI3K phosphorylation, it significantly upregulated Akt and eNOS phosphorylation in cardiomyocytes (Qi et al., 2023). Additional studies found that the PI3K/Akt pathway is closely linked to various diseases, including metabolic syndrome, gastrointestinal disorders, and allergies. Probiotic lactobacilli, such as L. rhamnosus GG, have been shown to modulate the PI3K/Akt pathway (Mohseni et al., 2021). However, probiotic lactobacilli increasing eNOS phosphorylation via PI3K/Akt pathway activation in human endothelial cells has yet to be established. In this study, we demonstrate that LpK79 activates the PI3K/Akt pathway, directly affecting eNOS activation in HUVECs.
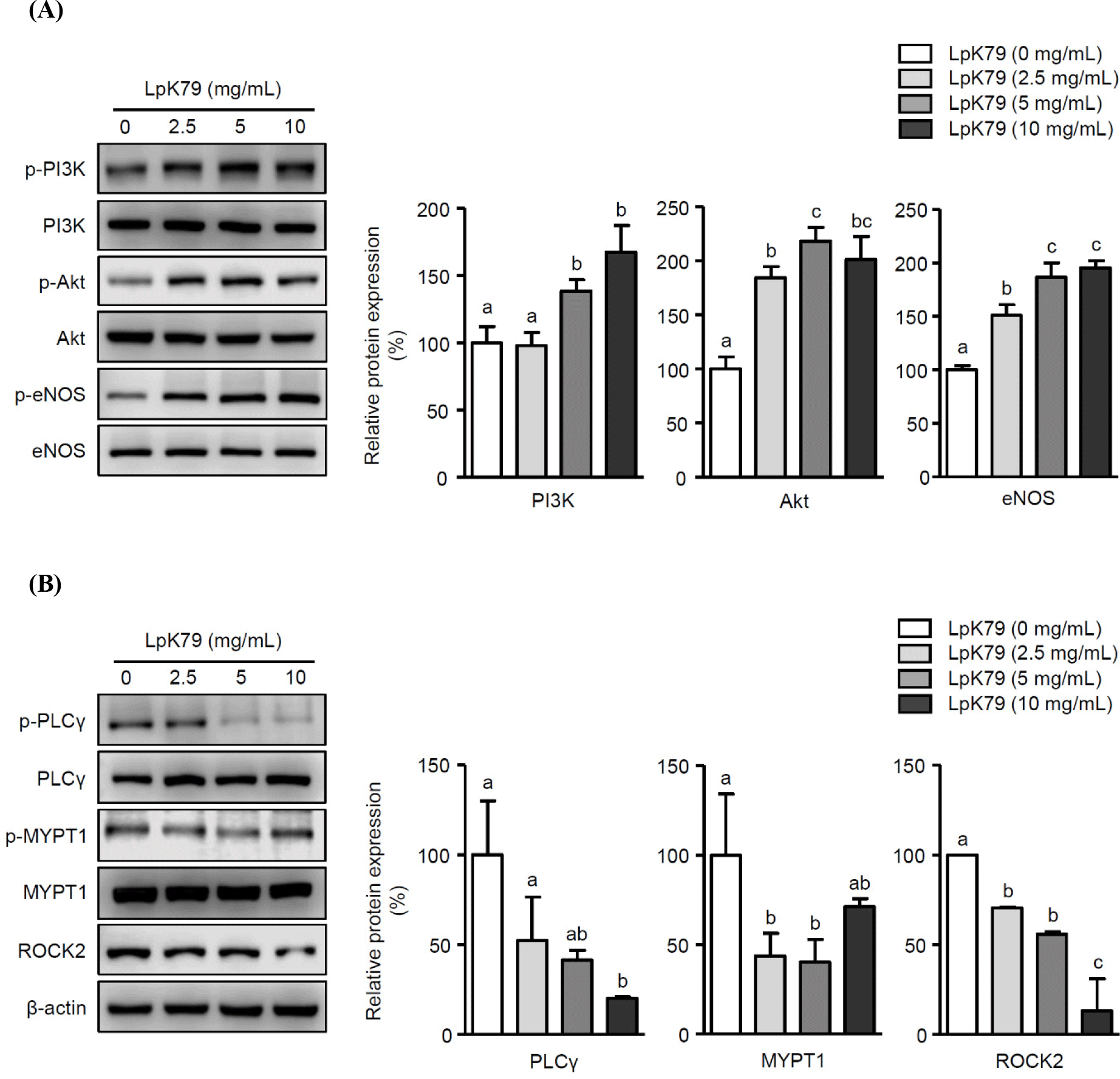
In addition to endothelial dysfunction, VSMC contractions contribute to hypertension (Yang and Hori, 2021). The ROCK pathway is crucial, and the myosin phosphatase target subunit (MYPT-1), an essential subset of ROCK targets, participates in VSMC contraction. Additionally, phospholipase Cγ (PLCγ) is integral for promoting VSMC contractions by increasing the intracellular calcium concentration (Touyz et al., 2018). Therefore, PLCγ and MYPT1 phosphorylation and ROCK2 activation in VSMCs were exposed to LpK79 to explore its impact on VSMC contraction. Notably, LpK79 treatment significantly decreased PLCγ and MYPT1 phosphorylation and ROCK2 expression (Fig. 2B). Calcium-independent MLC kinase (MLCK) and calcium-dependent MLC phosphatase (MLCP) pathways regulate MLC phosphorylation in smooth muscle cell contraction. The RhoA/ROCK signaling pathway, a calcium-independent MLCK pathway, activates MYPT1 phosphorylation, prompting MLC activation (Zhao et al., 2021). Additionally, PLC phosphorylation in the calcium-dependent MLCP pathway increases intracellular calcium ions, enhancing MLC phosphorylation and promoting VSMC contraction (Ma et al., 2023). Lactobacillus fermentum CECT5716 demonstrated antihypertensive effects in rats with NO synthase inhibition through vasodilation (Gómez-Guzmán et al., 2015). However, the intracellular mechanisms underlying VSMC contraction associated with probiotic lactobacilli remain unclear. Our study indicates that LpK79 is essential for regulating essential factors linked to VSMC contraction.
Impaired eNOS activation reduces NO production in HUVECs, resulting in endothelial dysfunction, such as inflammation. Thus, we examined whether LpK79 attenuated LPS-induced inflammatory responses in HUVECs. As illustrated in Fig. 3A, E. coli LPS augmented IL-6 and IL-8 mRNA expression. In contrast, LpK79 treatment significantly reduced IL-8 mRNA expression but not IL-6 mRNA. However, LpK79 significantly diminished IL-6 secretion in E. coli LPS-induced HUVECs and IL-8 secretion. These results suggest that LpK79 suppresses endothelial dysfunction-related inflammatory responses. IL-6 impairs endothelial barrier function and elevates angiotensin II type 1 receptor expression (Wassmann et al., 2004). Angiotensin II is an endocrine ligand that contributes to hypertension by interacting with its angiotensin II type 1 receptor (Eguchi et al., 2018). Therefore, a high IL-6 level in HUVECs has a positive influence on the interaction between angiotensin II and angiotensin II type 1 receptors, increasing hypertension risk. While our observations indicated that IL-6 mRNA expression in HUVECs was not significantly reduced when exposed to LpK79, there was an effective suppression of IL-6 protein levels. This discrepancy may be because mRNA expression was assessed in HUVECs for an extended duration of 24 h. In contrast, IL-8 mRNA and protein levels were significantly suppressed in HUVECs with LpK79 at 24 h. IL-8 is also closely related to the vascular inflammatory process, and excessive IL-8 production was observed in atherosclerotic lesions (Papadopoulou et al., 2008). Thus, inhibiting IL-8 and other inflammatory mediators in activated HUVECs could be beneficial in maintaining endothelial homeostasis and mitigating the risk of vascular diseases.
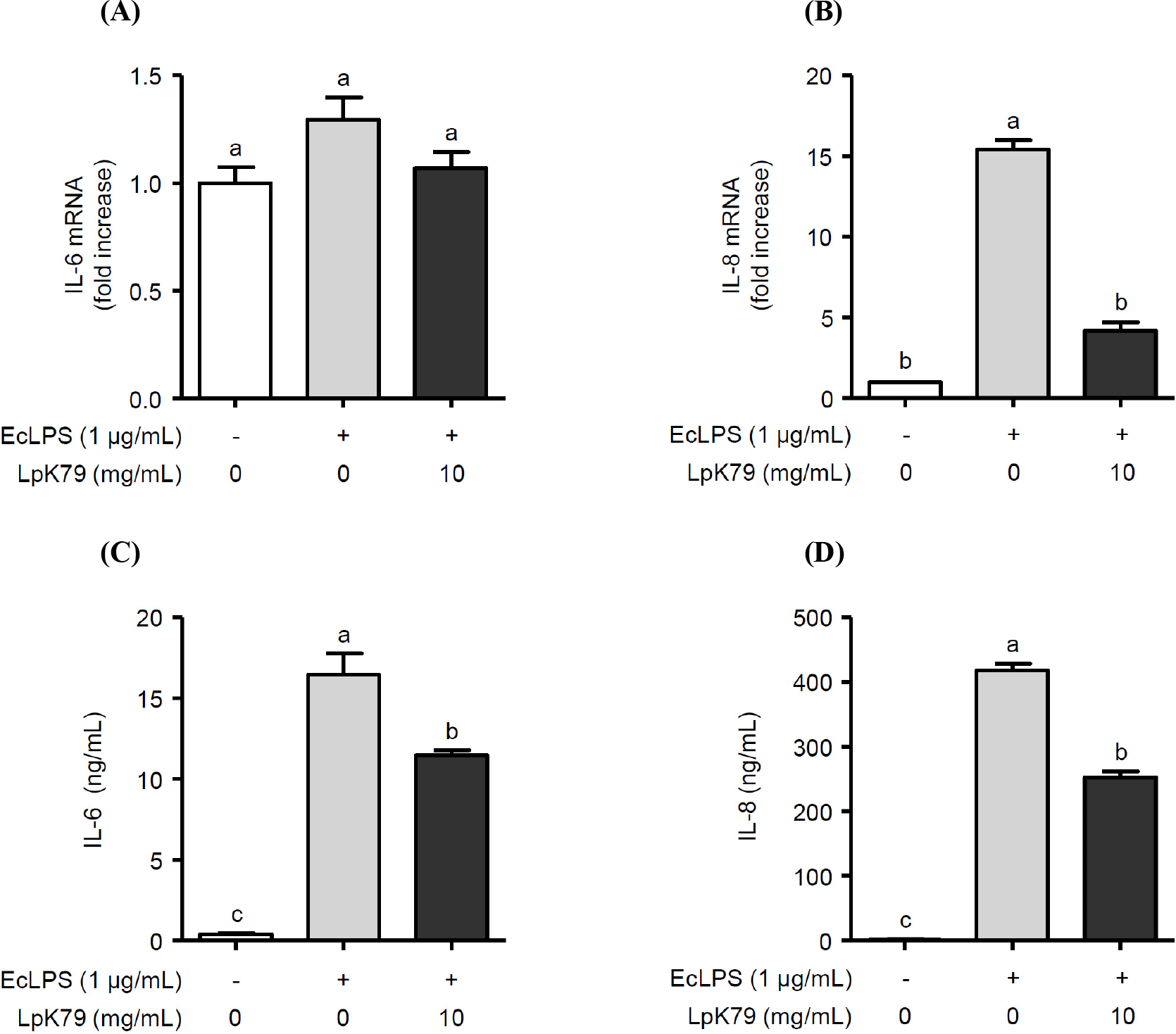
Conclusion
This study demonstrated that LpK79 increased NO production by activating eNOS through the PI3K/Akt pathway in HUVECs while reducing the expression of factors associated with VSMC contraction. Additionally, LpK79 suppressed inflammatory mediator expressions in HUVECs. These results suggest that LpK79 can provide a beneficial effect on the regulation of vascular endothelial function (Fig. 4).
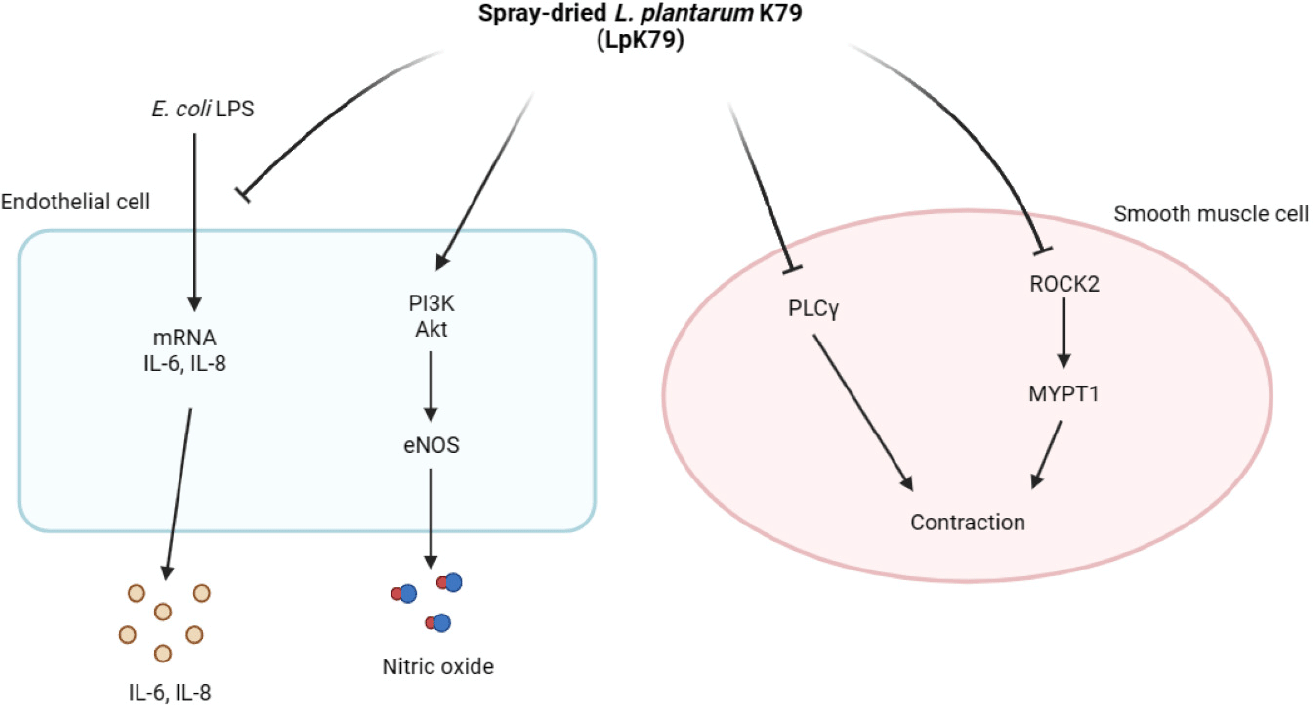