Introduction
Millions of sows are raised for breeding globally and each carries, on average, three to five litters. However, if continuous production becomes impossible or breeding fails, sows are culled (Bergman et al., 2018; Rodriguez-Zas et al., 2003; Sindelar et al., 2003). Up to 50% (or over 3 million) of breeding sows on many farms are culled annually (Blair and Lowe, 2019; USDA, 2016). Currently, commercial pigs are distributed in the market and produce excellent quality meat due to the control of feeding adjustments, age, and the rearing environment of these animals. Meat producers have a perception that the quality of meat from cull sows will be lower than that of commercial pigs due to their breeding environment (Sindelar et al., 2003). In South Korea, meat from culled sows is mainly distributed to the processed market rather than sold fresh due to their inappropriate appearance and dark red color (Hoa et al., 2020). However, little information is available regarding the meat quality and processing suitability. Therefore, research on raw materials will provide important information to consumers and processors.
The feeding system, age, and rearing environment have various effects on meat quality characteristics. Commercial pigs produced for meat production are slaughtered at approximately 180 days, representing the point of maximum profit, and have an average body weight of about 120 kg. Cull sows are maintained for a longer period to produce litters; their average body weight must be maintained at 160–180 kg or more due to allow adequate fetal growth and lactation (Aherne et al., 1999; Williams et al., 2005). In addition, cull sows experience different feeding regimes to commercial pigs, such as long-term feed intake, the restriction of feeding during pregnancy, and receiving the supplementary feed. In addition to their weight and age, all breeding practices of cull sows differ from those used for commercial pigs. This manifests as changes in the resultant pork quality (Huff-Lonergan and Lonergan, 2005; Mancini and Hunt, 2005). For example, older and heavier pigs develop darker, reddish flesh (Latorre et al., 2004). A higher pH and less drip loss are also encountered in pigs with weight gain (Virgili et al., 2003) and restricted feeding limits carcass and intramuscular fat accumulation, resulting in the reduced tenderness and juiciness of pork products (Lebret et al., 2001). In addition, various factors such as sex, breed, amount of exercise, stress, and nutritional status can affect pork quality (Rosenvold and Anderson, 2003). Therefore, the quality characteristics and chemical composition of meat obtained from cull sows can be expected to be vastly different to those of pork from commercial pigs.
Nutritional, sensory, and technological characteristics of meat are important factors in determining its quality. Research into meat quality is important in the meat processing industry and also to consumers when selecting products. Therefore, an investigation of the chemical composition and quality characteristics of pork obtained from cull sows will provide helpful information in this regard, increase value, and suggest utilization plans. In addition to research on the general quality of cull sow pork, it is crucial to investigate the fundamental factors that determine the standard of sows and elucidate its relationship with meat quality characteristics. Muscle fiber characteristics are related to meat grade (Jeong et al., 2010; Ryu and Kim, 2006) as they form the skeletal musculature that constitutes 75%–92% of meat. The muscle fibers of skeletal muscles are generally classified into type I, IIA, IIX, and IIXB, each displaying unique metabolic characteristics (Schiaffino and Reggiani, 1996). The muscle fiber type composition may change depending on various factors such as breed (Ryu and Kim, 2006), sex (Ozawa et al., 2000), age (Li et al., 2020), diet (Jeong et al., 2012), and muscle location (Hwang et al., 2010). In turn, the individual characteristics of muscle fiber types affect meat characteristics such as meat color, water-holding capacity, and texture (Pette and Staron, 2000). Therefore, changes in the quality characteristics of sows can be explained through an investigation of associated muscle fiber attributes; if sows display excellent quality characteristics, their muscle fibers can provide information to serve as a guideline in meat quality improvement.
The processed meat market has become as important as the fresh meat market due to the increasing needs and satisfaction of a growing consumer base. In general, non-preferred carcass parts such as hind legs are preferred for consumption over fresh meat (Vandendriessche, 2008). This is an attractive factor for processors, as it can lower the cost of meat products and simultaneously utilize non-preferred body parts. Most cull sows are distributed to the processing market, and their hind legs are typically used (Sindelar et al., 2003). However, such meat product manufacturing using non-preferred cuts focuses on hard-to-consume parts which are processed regardless of the functional characteristics of the raw meat (Troy and Kerry, 2010). Raw meat with poor technical characteristics such as water-holding capacity can significantly affect product yield, nutrition, texture, and color during processing due to water loss (Oh et al., 2008). Therefore, investigating the quality characteristics of raw meat will provide a means to confirm its suitability for processing.
In this study, we hypothesized that the environmental factors experienced by cull sows and commercial pigs prior to slaughter can cause differences in their quality characteristics and muscle fiber composition, ultimately leading to a change in processing aptitude. Therefore, this study compared the quality characteristics, chemical composition, and muscle fiber characteristics of the three major muscles of cull sow and commercial pig hind legs, analyzing the relationship between the quality characteristics and muscle fiber attributes. In addition, we aimed to link pork processing suitability with the quality attributes of fresh meat from cull sows to assess its applicability.
Materials and Methods
Commercial pigs [(Landrace×Yorkshire) ××Duroc ♂, LYD or (Yorkshire×Landrace) ×Duroc ♂, YLD species] and cull sows (Landrace×Yorkshire, LY or Yorkshire×Landrace, YL species) were purchased 48 hours post-mortem from the local market. The commercial pigs were generated by breeding 20 Duroc with 300 LY or YL. The pigs were in the same feeding condition according to the Korean Feeding Standard for Swine (RDA, 2022). A total of 40 pigs were used in the experiment; 20 commercial pigs and 20 cull sows were selected randomly. Their hot carcasses were then graded according to the standard grading procedure of the Korea Institute of Animal Products Quality Evaluation (KAPE, 2022). The carcass grade, backfat, and carcass weight are shown in Table 1. The commercial pigs possessed an average carcass weight of 83–92 kg, while that of cull sows was 183–195 kg. Ten hind legs of cull sows and commercial pigs (raised on different farms) were purchased at intervals of 7 d. Three representative hind leg muscles (biceps femoris, semimembranosus, semitendinosus) were selected for comparative purposes. Immediately after the purchase of the hind legs, these muscles were isolated, refrigerated at 4°C for 24 h, and used for experiments. For the investigation of flesh color, pH, and muscle fiber characteristics, whole muscles were used after removing all connective tissue and fat. For the analysis of the proximate composition, water-holding capacity (drip loss and cooking loss), total collagen content, and protein solubility, each muscle was pulverized into 6 mm particles and sufficiently mixed to obtain a uniform sample. In order to measure muscle fibers, samples were taken from the central part of the biceps femoris, semimembranosus, and semitendinosus and stored frozen in liquid nitrogen. The entire experimental process is shown in Fig. 1.
Moisture, crude protein, and crude ash content were analyzed for proximate composition using the AOAC (2000) method with pulverized cull sow and commercial pig muscle samples. Moisture content was expressed as a percentage (%) of the weight of a sample before drying, obtained by measuring the sample weight after placing it in a drying oven at 102°C for 24 h. Crude protein content was determined using the Kjeldahl method. Crude ash content was measured via a classical dry-ashing technique at 550°C. Crude fat content was measured using the methodology of Folch et al. (1957). Moisture, crude protein, crude ash, and crude fat content were measured four times for each sample to obtain average values.
Three hind leg muscles of sows and commercial pigs were exposed to air for 10 min before measuring their color using a chroma meter (CR-400, Minolta, Tokyo, Japan) that was calibrated with a standard white plate (Y=93.5, X=0.3132, and y=0.3198). Measurements were repeated eight times in the central part of each sample. The measuring conditions were D65 illuminant, 2° standard observer, and 65 mm aperture. According to the Commission International de l’Eclairage (CIE) system, color was expressed as CIE L*, CIE a*, CIE b*, chroma, and hue angle (h°). The chroma and hue angle (h°) was calculated as (a*2+b*2)0.5 and tan−1 (b*/a*), respectively.
After mixing 30 g of a minced muscle sample with 270 mL of distilled water, it was homogenized for 30 s using a Polytron homogenizer (T25 basic, IKA Labortechnik, Selangor, Malaysia). All homogenized samples were analyzed with a pH meter (MP230, Mettler-Toledo, Greifensee, Switzerland). Four pH measurements were recorded for each sample, and the average value was used. The pH meter was calibrated at 20°C using three standard buffers of pH 4.0, 7.0, and 9.0.
Two methods were employed to assess the water-holding capacity of samples via weight loss assessments: drip loss and cooking loss measurements. Since the hind legs of pigs are mainly processed into ground meat products, we used ground samples that were shaped into patties to imitate a similar scenario. Eighty grams of a minced muscle sample was placed in a petri dish (90×15 mm) to form a patty, then placed on soaking pad and placed in a sealed container. Its weight was measured after storing a sample for 48 h at 4°C. Four replicates were taken for each sample, and the average value was used. The drip loss value (%) was calculated using the following Eq. (1):
For the cooking loss measurement, patties of the same type as described above were placed in a convection steam oven (RCO-050E, KOSTEM, Gwangju, Korea) and cooked at 80°C for 30 min before their weights were measured. Four measurements were taken for each sample, and the average value was used. The cooking loss value (%) was calculated using the following Eq. (2):
Total collagen content was determined by modifying the method of Kolar (1990) and expressed as mg/g. Four grams of a sample was put in an Erlenmeyer flask, 30 mL of 7 N sulfuric acid was added, and the mixture was heated at 105°C for 16 h on a heating plate (HP630D, Misung Scientific, Seoul, Korea). After hydrolysis, distilled water was added to a level of 500 mL, and the mixture was filtered using Whatman No. 1 filter paper. We combined 2 mL of the extract with 1 mL of chloramine T solution (1.41 g chloramine T, 10 mL distilled water, 10 mL n-propanol, and 80 mL citric buffer at pH 6), and the product was allowed to stand for 20 min. Absorbance was measured at 558 nm, and hydroxyproline content was determined from a standard curve. Collagen content was calculated from hydroxyproline content using the coefficient 7.25. Three measurements were taken for each sample, and the average value was used.
Protein solubility was measured according to the procedures suggested by Warner et al. (1997). For sarcoplasmic protein solubility, 3 g of the sample was mixed with 30 mL 25 mM potassium phosphate buffer (pH 7.2) and then homogenized for 30 s using a Polytron homogenizer. The homogenate was left at 4°C for 20 h, centrifuged at 2,600×g for 30 min, and centrifuged homogenate was filtered with Whatman No. 1 filter paper. We measured the protein concentration of the filtered extract using the Biuret method with a bovine serum albumin standard curve. Total protein solubility was measured by mixing 3 g of a sample with 0.55 M potassium iodide and 50 mM potassium phosphate buffer (pH 7.2), with subsequent extraction conducted in the same way as described for sarcoplasmic protein solubility. The concentration of myofibrillar protein was calculated by subtracting the sarcoplasmic protein from total protein content. Three measurements were taken for each sample, and the average value was used.
The staining cross-sections of porcine skeletal muscles and classification of muscle fiber types were conducted according to Song et al. (2020b) with some modifications. Briefly, cross sections (10 μm thickness) were obtained from muscle cubes using a cryostat microtome (CM1520, Leica Biosystems, Wetzlar, Germany). Sections were blocked with 10% normal goat serum (Cell Signaling Technology, Danvers, MA, USA) for 1 h at room temperature. Primary antibodies (Developmental Studies Hybridoma Bank, Iowa City, IA, USA) were used to detect one or more myosin heavy chain isoforms (BA-F8, slow/I; SC-71, 2a and 2x; BF-35, all isoforms except for 2x; BF-F3, 2b). For multicolor immunofluorescence, Alexa Fluor 350, 488, and 594 (Thermo Fisher Scientific, Waltham, MA, USA) were applied to each section for 1 h at room temperature. Primary and secondary antibodies were applied sequentially and in cocktails to sections. After incubation, all sections were rinsed thrice for 5 min with phosphate‐buffered saline. All muscle fibers were inspected with a confocal scanning laser microscope (TCS SP8 STED, Leica Biosystems). Muscle fiber types were identified and classified into five types (I, IIA, IIX, IIXB, and IIB) according to the distribution of myosin heavy chain isoforms. Eighteen muscle fibers from each region were analyzed, and muscle fiber number composition (%), fiber area composition (%), and cross-sectional area (μm2) were measured using Image-Pro Plus software (Media Cybernetics, Rockville, MD, USA). Three measurements were taken for each sample, and the average value was used.
All experiments were performed in the same location. A total of 20 cull sows and 20 commercial pigs were analyzed in batches of 10 pigs at 7 d intervals. Twenty-five dependent variables and one independent variable were used, and a general linear model was used to test the pig breed effect. The muscle fibers of six pigs were analyzed during the same period. Each variable was repeatedly measured (three times or more) and averaged for comparative purposes. All results are expressed as the mean±SE. Data analyses were conducted with ANOVA procedure SAS version 9.3 (SAS Institute, Cary, NC, USA). The mean values in differences of quality characteristics between pig groups were confirmed through Duncan’s multiple range test at the 95% significance level.
Results and Discussion
Table 2 shows the moisture, crude protein, crude fat, and crude ash content of three major hind leg muscles of cull sows and commercial pigs. There was no significant difference in these attributes between the muscle groups of cull sows and commercial pigs (p>0.05). This corresponds with the results of Song et al. (2020a). In contrast, Hoa et al. (2020) and Kim and Kim (2018) reported significant differences in the crude protein and moisture content of a similar comparison. The differences were speculated to be attributed to the increased age of sows, genetic factors, or variations in feeding methods. However, most of these studies using cull sows analyzed a variety of hind leg muscles, leading to methodological differences. Based on the results of this study, it is determined that other growth environments (e.g., age, genetic factors, and feeding methods) of the sow did not affect the proximate composition changes of the three muscles (biceps femoris, semimembranosus, semitendinosus).
Table 3 summarizes the colors observed for the three muscles we investigated in cull sows and commercial pigs. Cull sows possessed a lower CIE L* and hue angle in the biceps femoris, semimembranosus, and semitendinosus (p<0.05); higher CIE a* in all muscles (p<0.05); and higher chroma in the biceps femoris and semimembranosus (p<0.05). Consumers generally assess the freshness of meat based on its color to decide on purchase (Forbes et al., 1974). In addition, color is used as a means of predicting meat quality through values of CIE L*, CIE a*, CIE b*, chroma, and hue angle (Hughes et al., 2014; Norman et al., 2003). The hue angle value changes according to the color (from red to yellow), a larger angle indicates less red pigmentation, and a higher chroma value is associated with a more vivid color. Our results indicate that pork from cull sows displayed decreased CIE L*, increased CIE a*, and vivid color. Meat color varies depending on parameters such as pig breed, age, sex, motility, and muscle group (Forrest et al., 1975); after slaughter, it is further affected by packaging conditions, the aging process, and lipid oxidation during exposure to consumers when being displayed to consumers (Domínguez et al., 2019). Meat from cull sows in this study exhibited a dark and reddish color, as has been reported for pigs with an increased breeding period (Miao et al., 2009). Since these sows are bred over a long period, their meat develops a dark and reddish color due to increased age.
Table 4 shows the pH values recorded for the muscles of cull sows and commercial pigs. In cull sows, the pH was high in all muscles, the biceps femoris, semimembranosus, and semitendinosus (p<0.05). The pH of meat helps with predicting quality and is closely associated with moisture content (Huff-Lonergan and Lonergan, 2005), as pH is related to an electrical attraction that can maintain moisture in the meat. When the number of positively and negatively charged groups in proteins equalizes, the attraction force between proteins is maximized, and water escapes from the cells; at the isoelectric point (around pH 5.5), the sum of the total charges becomes zero, resulting in maximum moisture loss (Huff-Lonergan and Lonergan, 2005). In live animals, acid-base homeostasis maintains a pH of about 7.0–7.2 in muscle tissue (Tarrant et al., 1972), as determined by metabolic activity within the muscle (Kylä-Puhju et al., 2004). However, muscle tissue converts into meat during rigor mortis, at which point the muscles undergo anaerobic metabolic activity that causes a decrease in pH due to the production of lactic acid. The difference in anaerobic metabolism activity varies depending on the muscle fiber type. It is also associated with meat color. Muscle fiber type Type 2b, representing white muscle, induces more anaerobic metabolism activity, resulting in muscle pH differences. Cull sows and commercial pigs, with different colors, would have differences in muscle fiber type. Therefore, the difference in meat pH between commercial pigs and cull sows is considered to be influenced by muscle fiber type.
Table 4 records the drip loss values of the three muscle groups we investigated. The drip loss values did not differ significantly among any muscles (p>0.05); however, numerically, all muscle of sows was about 1%–2% lower than that of commercial pigs. Drip loss is reflected in the amount of water that escapes from meat without applying an external physical force, and it is closely related to the pH of the meat (Qiao et al., 2007). Since no external force is applied, electrical attraction among proteins is maintained via the pH to prevent the exudation of moisture (Huff-Lonergan and Lonergan, 2005). Cull sows with a high meat pH were expected to have less drip loss but showed no significant difference in this study. This may be related to muscle structure. Drip loss due to structural factors mainly involves the development of extracellular spaces and postmortem proteolysis (Bertram et al., 2004; Offer et al., 1989). In Fig. 2, the extracellular space between muscle fibers is considered to be wider in cull sows than in commercial pigs. This tendency reportedly causes high water loss, as the extracellular space provides a drip channel that conducts water to the surface of the meat (Offer et al., 1989). As a result, cull sows with a larger extracellular space were expected to experience more drip loss, but the high pH electrical force prevented moisture loss. Therefore, it is concluded that these two conditions counteracted each other, resulting in no change in drip loss.
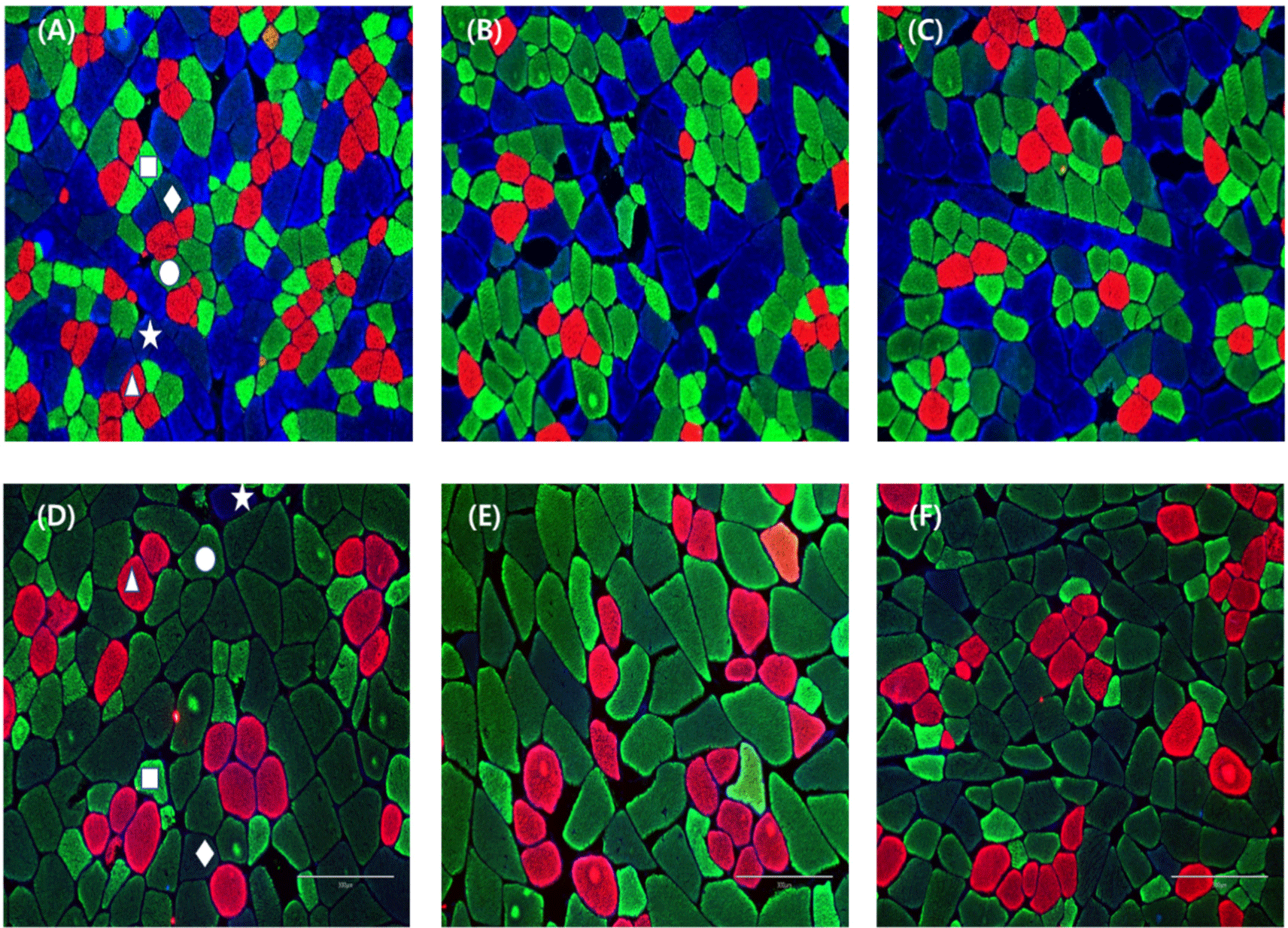
Table 4 shows the cooking loss values across three muscle groups. In cull sows, cooking loss was low in all muscles, the biceps femoris, semimembranosus, and semitendinosus (p<0.05). Cooking loss was determined by measuring the water liberated by applying heat to the meat. Most water loss during cooking is related to the denaturation of proteins and collagen in response to increased temperatures, causing a contraction of muscle fibers that allows water to escape (Lepetit et al., 2000). Protein denaturation, in particular, results in shrinkage of the meat and is one of the main causes of cooking loss (Tornberg, 2005). However, an investigation of protein and collagen content in this study found that their effect on the cooking yield was insignificant. Current research regarding cooking loss in meat remains inconclusive beyond the effects of protein and collagen. If other conditions (ex. muscle fiber characteristic) are responsible for cooking yield increases, cull sows can provide important information on pork quality. Therefore, the reduced cooking loss results in this study are considered to offer a new perspective beyond the well-known influence of collagen and protein, which have the strongest correlation with cooking loss.
Table 4 lists the protein solubility of cull sow and commercial pig meat across three muscle groups. There was no significant difference between cull sows and commercial pigs in the protein solubility recorded in all muscles (p>0.05). Despite this lack of statistical significance for protein solubility, it tended to be numerically higher in the semimembranosus and semitendinosus muscles of cull sows, indicating a degree of protein denaturation. Water content in meat is closely related to protein denaturation resulting from cooking and rigor mortis (Lopez-Bote et al., 1989). Most water is retained in the thin (actin) and thick (myosin) filaments of myofibrillar proteins. Actin and myosin, responsible for muscle relaxation and contraction, contract due to heat (Tornberg, 2005). The resultant contraction of the space holding water causes moisture loss. Any degradation of myofibrillar and cytoskeletal proteins is also associated with water loss. In addition, salt-soluble myofibrillar proteins play an important role in determining the quality characteristics of meat products (Santhi et al., 2017). Sarcoplasmic proteins affect attributes such as color and water-holding capacity (Sayd et al., 2006). Many studies have reported that sarcoplasmic proteins aggregate at 40°C–60°C and play a key role in the quality of processed meat (Farouk et al., 2002; Hamm, 1977). Protein in fresh and processed meat products determines quality. The close relationship between water loss and proteins may have influenced the results of water-holding capacity in this study. Our drip loss and cooking loss findings suggest that pork from cull sows possess a higher water-holding capacity than pork from commercial pigs, which could be partly explained by pH and protein solubility factors.
Table 4 displays the comparative total collagen content of three types of muscle from cull sows and commercial pigs, with no significant differences being recorded (p>0.05). Collagen, a stromal protein, forms the basic structure of connective tissue and plays a protective role in cells, muscles, tissues, organs, etc., by connecting or covering the exterior. In cooked meat, collagen produces a tough texture due to denaturation (Lewis and Purslow, 1989); water is exuded due to the contraction of the endomysial collagen fibers surrounding the water-bearing muscle fibers at a cooking temperature of 60°C–70°C (Lepetit et al., 2000). The total amount of collagen is proportional to the amount of muscle activity and varies for each muscle part (Hill, 1966). Although collagen content was expected to be increased in the hind legs of cull sows (due to their longer time of activity than commercial pigs), no such difference was observed. This study showed the opposite results to the general trend, in which meat from older animals lose more water during the cooking process (Shimokomaki et al., 1972). In this study, the effect of collagen on cooking loss did not seem as pertinent as that of pH, protein solubility, and muscle fiber characteristics.
Fig. 3 shows the muscle fiber number, area composition, and cross-sectional area present in cull sows and commercial pigs. The ratio of type I, type IIA, and type IIX fibers in the biceps femoris was high in cull sows, while the ratio of type IIB fibers was high in commercial pigs (p<0.05). In the semimembranosus muscle, the ratio of type IIB fibers was high in commercial pigs (p<0.05). In the semitendinosus muscle, the ratio of type I and type IIX fibers was high in cull sows, and the ratio of type IIA and type IIB fibers was high in commercial pigs (p<0.05). In terms of fiber area composition, the area ratio of type I, type IIA, and type IIX fibers in the biceps femoris was high in cull sows, while the area ratio of type IIB fibers was high in commercial pigs (p<0.05). In the semimembranosus, the area ratio of type I fibers was high in cull sows, while the area ratio of type IIB fibers was high in commercial pigs (p<0.05). In the semitendinosus muscle, the area ratio of type I and type IIX fibers were high in sows, and the area ratio of type IIA and type IIB fibers was high in commercial pigs (p<0.05). The cross-sectional area of type I, type IIA, type IIX, and type IIXB fibers in the biceps femoris was wider in cull sows than in commercial pigs (p<0.05). In the semimembranosus, the cross-sectional area of type I and type IIA fibers was also wider in cull sows (p<0.05). In the semitendinosus, the cross-sectional area of type I fibers was wider in cull sows, while the cross-sectional area of type IIA fibers was wider in commercial pigs (p<0.05).
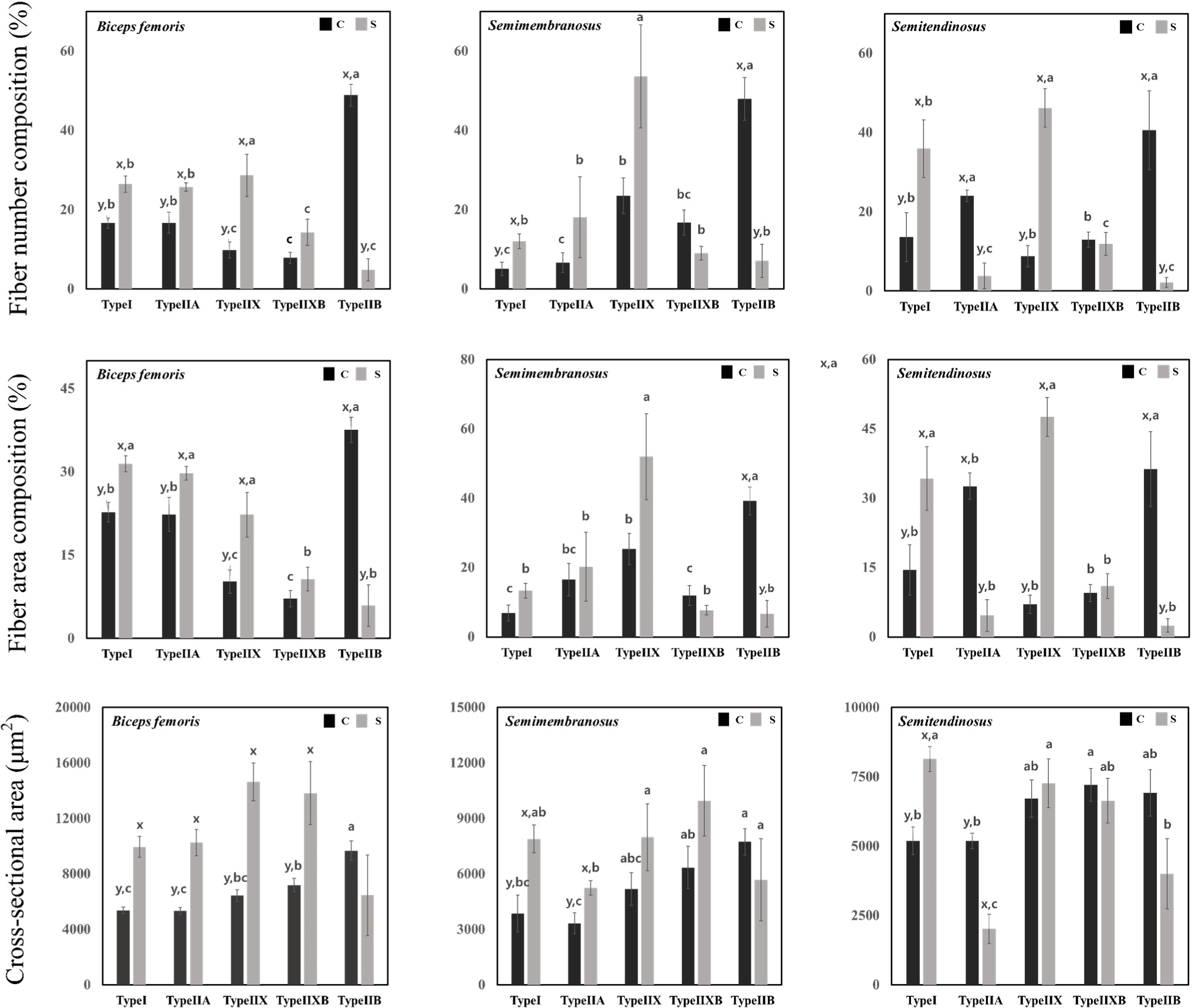
The types of muscle fibers include type IIB, type IIXB, type IIX, type IIA, and type I, each with different characteristics. Muscle fibers with different characteristics are broadly categorized into anaerobic metabolism and aerobic metabolism. Type IIX, type IIXB, and type IIB, Fast-twitch glycolytic fibers involved in anaerobic metabolism, perform glycolysis. Their metabolic activities play a role in reducing pH due to lactic acid accumulation. Type I and type IIA, involved in aerobic metabolism, participate in oxygen storage and transport, and they have higher pH compared to the muscle fibers involved in anaerobic metabolism. Due to the effect of these metabolic activities on muscle fibers, the presence of type IIB fibers is positively correlated with drip loss (Huff-Lonergan and Lonergan, 2005), and an increase in the ratio of type I fibers causes the color of the meat to become more red (Kim et al., 2010). Our findings suggest that an altered muscle fiber composition affected the metabolic activity and meat color in cull sows, causing an increase in pH and a red meat color. Furthermore, the change in muscle fiber composition, especially the increase in type I led to an increase in pH, which may have contributed to our results for water holding capacity. It is necessary to confirm the role of type IIX fibers, which account for 30%–50% of fiber number and area composition in cull sows. In general, type IIX fibers mediate the fiber type transition that occurs during growth and aging. Information on the characteristics of type I and type IIB is known, but the effect of type IIX on quality is known to play a role similar to that of type IIB (Klont et al., 1998). Although type IIX, which accounts for a large proportion in sows, is classified as a glycolytic muscle fiber, it does not seem to affect pH or water-holding capacity. Therefore, the effect of type IIX on quality is not regarded as significant.
Muscle fiber type composition may change over rearing period. In muscles that are involved in long-term endurance activities, muscle fibers may change from type IIB → type IIX(D) → type IIA → type I. Conversely, in muscles that require instantaneous force, fibers may change from type I → type IIA → type IIX(D) → type IIB (Caiozzo et al., 1992; Schiaffino and Reggiani, 1996); with an increase in age, they turn into endurance-requiring muscles, causing an increase in muscle fiber type I. Therefore, the observed increase in type I and the decrease in type IIB muscle fibers in cull sows may have occurred with an increase in age. The cross-sectional area of muscle fibers is also related to advancing age in carnivores; the total number of muscle fibers is genetically fixed, and only their lengths and cross-sectional areas increase over time (Stickland et al., 1975). We noted that the cross-sections of type I and IIA fibers similarly enlarged in older cull sows. However, it was not the increase in the cross-sectional area of the overall muscle fiber, but the size of the muscle fiber that increased in muscle fiber number and area composition. In other words, it was confirmed that the cross-sectional area did not increase in undeveloped muscle fibers.
Until now, studies on quality characteristics have been conducted according to muscle fiber types, but most studies related to muscle fiber size are related to texture. To our knowledge, no studies have investigated the cross-sectional area size and cooking characteristics of muscle fibers. However, since meat contracts while cooking, this process is highly related to the cross-sectional area of muscle fibers. Assuming that muscle fibers have the same density, an increase in the size of muscle fibers will mean that less physical deformation occurs during contraction, thereby retaining more water within the muscle fibers. We can conclude that the small cooking loss in cull sow meat observed in this study may be highly related to the size of muscle fibers.
Conclusion
Cull sows and commercial pigs show notable distinctions in muscle fiber type, size, and cross-sectional area due to their different growth environment. While these differences in muscle fibers don’t impact the pork’s chemical composition in cull sows, they do affect meat pH and color. The glycolytic muscle fiber type (type IIX) appeared to have little effect on meat quality. In addition, a small cooking loss was observed, which may result from an increase in the cross-sectional area of the muscle fibers. As a result, a difference in the quality of meat between the cull sow and the commercial pig was observed due to their different growth environments and resultant changes in muscle fiber characteristics, and cull sow was confirmed to provide meat suitable for cooking. More importantly, as a relatively low water loss was observed when cooking pork obtained from cull sows, this meat source can offer nutritional and economic advantages.